CHAPTER OUTLINE
Purine Nucleotide Biosynthesis
Regulation of Purine Nucleotide Synthesis
Catabolism and Salvage of Purine Nucleotides
Disorders of Purine Metabolism
Pyrimidine Nucleotide Biosynthesis
Regulation of Pyrimidine Biosynthesis
Catabolism of Pyrimidine Nucleotides
Formation of Deoxyribonucleotides
High-Yield Terms
5-phosphoribosyl-1-pyrophosphate (PRPP): activated form of ribose used in de novo synthesis of purine and pyrimidine nucleotides
Gout: a disorder that is related to excess production and deposition of uric acid crystals; does not occur in the absence of hyperuricemia
Severe-combined immunodeficiency (SCID): a disorder related to defects in the activity of the purine nucleotide catabolism enzyme, adenosine deaminase (ADA)
Tumor lysis syndrome (TLS): potentially fatal condition characterized by acute urate nephropathy, resulting from significant increases in purine nucleotide degradation from dying cancer cells
Nucleotide Metabolism
The metabolic requirements for the nucleotides and their cognate bases can be met by either dietary intake or synthesis de novo from low-molecular weight precursors. Indeed, the ability to salvage nucleotides from sources within the body essentially alleviates any nutritional requirement for nucleotides. Thus the purine and pyrimidine bases are not required in the diet. The hydrolysis and uptake of dietary nucleic acids is covered in Chapter 43. The salvage pathways are a major source of nucleotides for synthesis of DNA, RNA, and enzyme cofactors.
Both the de novo synthesis and salvage pathways of purine and pyrimidine nucleotide biosynthesis utilize an activated sugar intermediate: 5-phosphoribosyl-1-pyrophosphate (PRPP), which is generated by the action of PRPP synthetase (Figure 32-1).
FIGURE 32-1: PRPP synthetase reaction. Reproduced with permission of themedicalbiochemistrypage, LLC.
Purine Nucleotide Biosynthesis
The major site of purine synthesis is in the liver. Synthesis of the purine nucleotides begins with PRPP and leads to the first fully formed nucleotide, inosine 5′-monophosphate (IMP) (Figure 32-2). In purine nucleotide biosynthesis, the base is constructed upon the ribose by several amidotransferase and transformylation reactions. The synthesis of IMP requires 5 moles of ATP, 2 moles of glutamine, 1 mole of glycine, 1 mole of CO2, 1 mole of aspartate, and 2 moles of formate. The formyl moieties are carried on tetrahydrofolate (THF) in the form of N5,N10-methenyl-THF and N10-formyl-THF.
FIGURE 32-2: Purine biosynthesis from ribose 5-phosphate and ATP. (P, PO32−_ or PO2–.) Murray RK, Bender DA, Botham KM, Kennelly PJ, Rodwell VW, Weil PA. Harper’s Illustrated Biochemistry, 29th ed. New York, NY: McGraw-Hill; 2012.
IMP represents a branch point for purine biosynthesis because it can be converted into either AMP or GMP through 2 distinct reaction pathways (Figure 32-3). The pathway leading to AMP requires energy in the form of GTP; that leading to GMP requires energy in the form of ATP. The utilization of GTP in the pathway to AMP synthesis allows the cell to control the proportions of AMP and GMP to near equivalence. The accumulation of excess GTP will lead to accelerated AMP synthesis from IMP instead at the expense of GMP synthesis. Conversely, since the conversion of IMP to GMP requires ATP, the accumulation of excess ATP leads to accelerated synthesis of GMP over that of AMP.
FIGURE 32-3: Conversion of IMP to AMP and GMP. Murray RK, Bender DA, Botham KM, Kennelly PJ, Rodwell VW, Weil PA. Harper’s Illustrated Biochemistry, 29th ed. New York, NY: McGraw-Hill; 2012.
Regulation of Purine Nucleotide Synthesis
The essential rate-limiting steps in purine biosynthesis occur at the first 2 reactions of the pathway (Figure 32-4). The synthesis of PRPP by PRPP synthetase is feedback inhibited by purine-5′-nucleotides (predominantly AMP and GMP). Combinatorial effects of those 3 nucleotides are greatest, for example, inhibition is maximal when the correct concentration of both adenine and guanine nucleotides is achieved. The amidotransferase reaction catalyzed by PRPP amidotransferase is also feedback inhibited by binding ATP, ADP, and AMP at 1 inhibitory site and GTP, GDP, and GMP at another. Conversely, the activity of the enzyme is stimulated by PRPP. Additionally, purine biosynthesis is regulated in the branch pathways from IMP to AMP and GMP (Figure 32-5). The accumulation of excess ATP leads to accelerated synthesis of GMP and excess GTP leads to accelerated synthesis of AMP.
FIGURE 32-4: Control of the rate of de novo purine nucleotide biosynthesis. Reactions 1 and 2 are catalyzed by PRPP synthase and by PRPP glutamyl amidotransferase, respectively. Solid lines represent chemical flow. Broken red lines represent feedback inhibition by intermediates of the pathway. Murray RK, Bender DA, Botham KM, Kennelly PJ, Rodwell VW, Weil PA. Harper’s Illustrated Biochemistry, 29th ed. New York, NY: McGraw-Hill; 2012.
FIGURE 32-5: Regulation of the conversion of IMP to adenosine nucleotides and guanosine nucleotides. Solid lines represent chemical flow. Broken green lines represent positive feedback loops +, and broken red lines represent negative feedback loops . Abbreviations include AMPS (adenylosuccinate) and XMP (xanthosine monophosphate), whose structures are given in Figure 32–3. Murray RK, Bender DA, Botham KM, Kennelly PJ, Rodwell VW, Weil PA. Harper’s Illustrated Biochemistry, 29th ed. New York, NY: McGraw-Hill; 2012.
Catabolism and Salvage of Purine Nucleotides
Catabolism of the purine nucleotides leads ultimately to the production of uric acid (Figure 32-6), which is insoluble and is excreted in the urine as sodium urate crystals.
FIGURE 32-6: Pathways of purine nucleotide catabolism. Reproduced with permission of themedicalbiochemistrypage, LLC.
The synthesis of nucleotides from the purine bases and purine nucleosides takes place in a series of steps known as the salvage pathways (Figure 32-7). The free purine bases, adenine, guanine, and hypoxanthine, can be reconverted to their corresponding nucleotides by phosphoribosylation. The 2 key transferase enzymes that are involved in the salvage of purines are adenosine phosphoribosyltransferase (APRT) and hypoxanthine-guanine phosphoribosyltransferase (HGPRT). A critically important enzyme of purine salvage in rapidly dividing cells is adenosine deaminase (ADA), which catalyzes the deamination of adenosine to inosine.
FIGURE 32-7: Pathways of purine nucleotide salvage. ADA, adenosine deaminase; PNP, purine nucleotide phosphorylase. Reproduced with permission of themedicalbiochemistrypage, LLC.
Disorders of Purine Metabolism
The clinical problems associated with nucleotide metabolism in humans are predominantly the result of abnormal catabolism of the purines. The clinical consequences of abnormal purine metabolism range from mild to severe and even fatal disorders. Clinical manifestations of abnormal purine catabolism arise from the insolubility of the degradation byproduct, uric acid. Gout (Clinical Box 32-1) is a condition that results from the precipitation of urate as monosodium urate (MSU) or calcium pyrophosphate dihydrate (CPPD) crystals in the synovial fluid of the joints, leading to severe inflammation and arthritis.
Two severe disorders are associated with defects in purine metabolism: Lesch-Nyhan syndrome (Clinical Box 32-2) and severe combined immunodeficiency disease (SCID) (Clinical Box 32-3). Lesch-Nyhan syndrome results from the loss of a functional HGPRT gene and SCID is most often (90%) caused by a deficiency in the enzyme adenosine deaminase (ADA). The glycogen storage disease, von Gierke disease, (see Clinical Box 14-1) also leads to excessive uric acid production and gouty symptoms.
Pyrimidine Nucleotide Biosynthesis
The synthesis of pyrimidine nucleotides is less complex than that of the purines since the base is chemically much simpler (Figure 32-8). The first completed pyrimidine base (orotate monophosphate, OMP) is derived from 1 mole of glutamine, 1 mole of ATP, 1 mole of CO2, and 1 mole of aspartate. An additional mole of glutamine and ATP are required in the conversion of UTP to CTP. The synthesis of pyrimidines differs in 2 significant ways from that of purines. First, the ring structure is assembled as a free base, not built upon PRPP. PRPP is added to the first fully formed pyrimidine base (orotic acid), forming orotate monophosphate (OMP), which is subsequently decarboxylated to UMP. Second, there is no branch in the pyrimidine synthesis pathway. UMP is phosphorylated twice to yield UTP (ATP is the phosphate donor). The first phosphorylation is catalyzed by uridylate kinase and the second by ubiquitous nucleoside diphosphate kinase. Finally UTP is aminated by the action of CTP synthase, generating CTP.
FIGURE 32-8: The biosynthetic pathway for pyrimidine nucleotides. Murray RK, Bender DA, Botham KM, Kennelly PJ, Rodwell VW, Weil PA. Harper’s Illustrated Biochemistry, 29th ed. New York, NY: McGraw-Hill; 2012.
The de novo pathway to deoxythymidine triphosphate (dTTP) synthesis first requires the use of dUMP from the metabolism of either UDP or CDP. The dUMP is converted to dTMP by the action of thymidylate synthase. The unique property of the action of thymidylate synthase is that the tetrahydrofolate (THF) cofactor (N5, N10-methylene-THF) is converted to dihydrofolate (DHF), the only such reaction yielding DHF from THF. In order for the thymidylate synthase reaction to continue, THF must be regenerated from DHF. This is accomplished through the action of dihydrofolate reductase (DHFR). The crucial role of DHFR in thymidine nucleotide biosynthesis makes it an ideal target for chemotherapeutic agents (see later). The salvage pathway to dTTP synthesis involves the enzyme thymidine kinase, which can use either thymidine or deoxyuridine as substrate. The activity of thymidine kinase is unique in that it fluctuates with the cell cycle, rising to peak activity during the phase of DNA synthesis.
CLINICAL BOX 32-1: HYPERURICEMIA AND GOUT
Gout is a disorder that is related to excess production and deposition of uric acid crystals. The root cause of gout is hyperuricemia and it is characterized by recurrent attacks of acute inflammatory arthritis. The formation of urate crystals leads to tophaceous deposits (sandy, gritty, nodular masses of urate crystals), particularly in the joints, which precipitates the episodes of gouty arthritis. Gouty arthritis is the most painful manifestation of gout and is caused when urate crystals interact with neutrophils, triggering an inflammatory response. Gout does not occur in the absence of hyperuricemia. Hyperuricemia is defined as a serum urate concentration exceeding 7.0 mg/dL in men and 6.0 mg/dL in women. Hyperuricemia can result from either excess uric acid production or reduced excretion or a combination of both mechanisms. Primary gout is a biochemically and genetically heterogeneous disorder resulting from inborn metabolic errors that alter uric acid homeostasis. There are at least 3 different inherited defects that lead to early development of severe hyperuricemia and gout: glucose-6-phosphatase deficiency; severe and partial HGPRT deficiency; and elevated PRPP synthetase activity. At least 3 different isoforms of PRPP synthetase have been identified and are encoded by 3 distinct, yet highly homologous genes, identified as PRPS1, PRPS2, and PRPS3. The PRPS1 and PRPS2 genes are found on the X chromosome and the PRPS3 gene is found on chromosome 7. Mutations in the PRPS genes that result in superactivity lead to enhanced production of PRPP. Increased levels of PRPP, in turn, drive enhanced purine nucleotide synthesis in excess of the needs of the body. Thus, the excess purine nucleotides are catabolized, resulting in elevated production of uric acid and consequent hyperuricemia and gout. A complete or virtually complete loss of HGPRT activity results in the severe disorder, Lesch-Nyhan syndrome (Clinical Box 32-2). Deficiencies in glucose-6-phosphatase result in von Gierke disease (Clinical Box 14-1), yet associated with this defect is increased uric acid production and symptoms of gout. Hyperuricemia does not always lead to the typical clinical manifestations of gout. These symptoms usually only appear in a person suffering with hyperuricemia for 20 to 30 years. The normal course of untreated hyperuricemia, leading to progressive urate crystal deposition, begins with uric acid urolithiasis (urate kidney stones) and progresses to acute gouty arthritis, then intercritical gout and chronic tophaceous gout. Intercritical gout refers to the period between gouty attacks. Diagnosis of gout during these periods is difficult, but analysis for urate crystals in the synovial fluids of a previously affected joint can establish a correct diagnosis. Numerous circumstances such as trauma, surgery, excessive alcohol consumption, administration of certain drugs, and the ingestion of purine-rich foods can precipitate gouty attacks. Acute gouty arthritis consists of painful episodes of inflammatory arthritis and represents the most common manifestation of gout. Typical descriptions of this symptom include a patient who goes to bed and is awakened by severe pain in the large toe, which may also be experienced in the heel, instep, or ankle. The pain can become so severe that a simple act of cloth touching the area becomes unbearable. Gouty arthritis attacks usually dissipate within several hours, but can also last for several weeks. The inflammatory processes initiated in gouty arthritis are the result of neutrophil ingestion (phagocytosis) of urate crystals and the subsequent release of numerous inflammatory mediators. Chronic tophaceous gout results after a number of years in an untreated individual. Episodes of acute gouty arthritis become more frequent and severe and the intercritical period may disappear completely. This stage of the disease is characterized by the deposition of solid urate (tophi) in the articular cartilage and other connective tissues. The continued development of tophi results in destructive arthropathy (disease of a joint). Aside from gouty arthritis and tophus formation, renal disease is the most frequent complication of hyperuricemia. Kidney disease in patients with gout is of numerous types. Urate nephropathy is the result of the deposition of monosodium urate crystals in the renal interstitial tissue. Uric acid nephropathy is caused by the deposition of uric acid crystals in the collecting tubules, renal pelvis, or the ureter and results in impaired urine flow. Calcium oxalate urolithiasis also occurs in hyperuricemic patients. Uric acid urolithiasis (uric acid kidney stones) accounts for approximately 10% of all urinary calculi (stones) in the United States. Treatments aimed at lowering serum urate levels in hyperuricemic patients is usually only a consideration in the context of gout. Because acute gouty arthritis is an inflammatory event, treatment with anti-inflammatory drugs is often successful in reducing the symptoms. The most commonly prescribed drug for reducing the production of uric acid is the xanthine oxidase inhibitor, allopurinol.
CLINICAL BOX 32-2: LESCH-NYHAN SYNDROME
Lesch-Nyhan syndrome (LNS) is a disorder related to defects in the activity of HGPRT. HGPRT catalyzes the interconversions of hypoxanthine and IMP and guanine and GMP. There are 3 overlapping clinical syndromes associated with deficiencies in HGPRT activity. Individuals that have less than 1.5% residual enzyme activity exhibit debilitating neurologic disability, behavioral abnormalities that include impulsive and self-mutilating behaviors, and varying degrees of cognitive disability in addition to overproduction of uric acid. This most severe of the 3 clinical syndromes is LNS. Patients with 1.5% to 8% of residual enzyme activity exhibit neurologic disability that ranges from clumsiness to debilitating pyramidal (CNS neurons involved in voluntary motor movement) and extrapyramidal motor dysfunction in addition to overproduction of uric acid. In cases where at least 8% of normal HGPRT activity is present, patients exhibit overproduction of uric acid and associated hyperuricemia, renal lithiasis, and gout. The latter circumstance (partial deficiency with at least 8% enzyme activity) is commonly referred to as Kelley-Seegmiller syndrome. Lesch-Nyhan syndrome is inherited as an X-linked recessive disorder with an incidence of approximately 1:380,000. Since it is an X-linked disease, it is found almost exclusively in males although affected females have been identified, albeit very rarely. The characteristic clinical features of the LNS are mental retardation, spastic cerebral palsy, choreoathetosis, uric acid urinary stones, and self-destructive biting of fingers and lips. The overall clinical features of LNS can be divided into 3 broad categories. These include uric acid overproduction and its associated consequences (eg, gouty arthritis and renal lithiasis), neurobehavioral dysfunction indicative of central nervous system involvement, and growth retardation. All patients with LNS manifest with profound motor dysfunction that is recognizable within the first 3 to 9 months of life. Infants fail to develop the ability to hold up their heads or to sit unaided. Further motor development will be delayed and the onset of pyramidal and extrapyramidal signs becomes evident by 1 to 2 years of age. In LNS patients there are 3 major signs of pyramidal dysfunction: spasticity, hyperreflexia, and the extensor plantar reflex (also known as the Babinski reflex). Extrapyramidal dysfunction in LNS patients is primarily evident as dystonia (sustained muscle contractions causing twisting and repetitive movements or abnormal postures), although many patients also exhibit choreoathetosis (involuntary movement disorder in association with slow continuous writhing particularly of the hands and feet). Most commonly associated with LNS is the behavioral dysfunction manifest with impulsivity and self-mutilation, particularly of the lips, fingers, and tongue. LNS patients will often strike out at people around them, spit on people, and use foul language. These symptoms are analogous to the uncontrollable compulsions associated with Tourette syndrome. The self-injury behavior is clearly an involuntary action as most LNS patients will learn to call out for help when they feel the compulsive behavior overtaking them, or they will sit on their hands or wear socks or gloves to limit the self-injurious behavior.
CLINICAL BOX 32-3: SEVERE COMBINED IMMUNODEFICIENCY SYNDROME, SCID
Severe combined immunodeficiency disease (SCID) is a disorder related to defects in the activity of adenosine deaminase (ADA). SCID is inherited as an autosomal recessive disorder with an incidence as high as 1:200,000. ADA catalyzes the irreversible deamination of adenosine and deoxyadenosine to inosine and 2′-deoxyinosine, respectively. Although ADA activity is found in all tissues of the body, the highest concentrations are found in the thymus and other lymphoid tissues. In addition to the differences in levels of ADA in various tissues, the enzyme is also developmentally regulated such that immature thymocytes express higher levels than do mature thymocytes. The level of expression of ADA also decreases as B cells mature. In ADA deficiency, there is an elevation in the level of adenosine and 2′-deoxyadenosine levels in the blood and urine are also elevated. The consequences of the elevations in these 2 ADA substrates are impaired lymphocyte differentiation, function, and viability, which results in lymphopenia and severe immunodeficiency. Increases in 2′-deoxyadenosine results in dramatic increases in cellular dATP pools. The consequence of increased dATP is an inhibition of ribonucleotide reductase (RR), the enzyme responsible for generating deoxyribonucleotides necessary for DNA replication. The inhibition of RR leads to a block in DNA replication that is critical for the expansion of lymphocytes in response to an antigenic challenge. Deoxy-ATP also induces DNA strand breakage in nondividing lymphocytes via a direct activation of caspase 9 involved in apoptosis. In addition, S-adenosylhomocysteine hydrolase activity is markedly inhibited by 2’-deoxyadenosine, resulting in accumulation of S-adenosylhomocysteine that in turn results in reduced synthesis of S-adenosylmethionine, a critical substrate in transmethylation reactions. Although not all forms of SCID are caused by defects in ADA, greater than 85% of all ADA-deficient patients are infants with SCID. Infants with SCID usually present within the first month of life with failure to thrive and life-threatening interstitial pneumonitis (inflammation of the walls of the airs sacs of the lungs). Pneumonia is often caused by Pneumocystis carinii or a viral infection. The gastrointestinal tract, as well as the skin, is also frequently involved in initial infections. Candidiasis is first observed as “diaper rash” but then progresses to an extensive infection involving the skin, oral, and esophageal mucosa and the vagina in female patients. Persistent infections prompt most parents to have their baby examined, and SCID is thus often diagnosed by 1 to 2 months of age from a determination of immune function. Most SCID patients lack both cell-mediated (T cell) and humoral (B cell) immunity, thus the derivation of the disease name: severe combined immunodeficiency. Because this disease is severely life-threatening, most patients do not survive for more than a few months. If immunological function is not restored, SCID is always fatal by 1 to 2 years of age. The most effective treatment for SCID is bone marrow transplantation. Enzyme replacement therapy (ERT) has proved effective in treating some (but not all) ADA-deficient SCID patients. The most common treatment is to use intramuscular injection of highly purified bovine ADA coupled to the inert polymer, monomethoxy polyethylene glycol (PEG-ADA). The use of PEG-ADA therapy is sometimes warranted in SCID patients who are too ill to undergo bone marrow transplantation.
CLINICAL BOX 32-4: TUMOR LYSIS SYNDROME
Chemotherapeutic treatment of leukemias, non-Hodgkin lymphomas, and Burkitt lymphomas often results in tumor lysis syndrome (TLS). TLS is caused by massive tumor cell lysis, with the release of large amounts of potassium, phosphate, and nucleic acids into the systemic circulation. Catabolism of the purine nucleic acids to uric acid leads to hyperuricemia. The dramatic increase in uric acid excretion can result in the precipitation of uric acid in the renal tubules and can also induce renal vasoconstriction, impaired autoregulation, decreased renal blood flow, and inflammation, referred to as acute urate nephropathy. Hyperphosphatemia with calcium phosphate deposition in the renal tubules can also cause acute kidney injury. Treatment of TLS includes aggressive hydration and diuresis, monitoring of electrolyte abnormalities, and control of hyperuricemia with allopurinol and rasburicase.
Regulation of Pyrimidine Biosynthesis
The regulation of pyrimidine synthesis occurs mainly at the first step catalyzed by aspartate transcarbamoylase (ATCase). ATCase is a multifunctional enzyme in mammalian cells, capable of catalyzing the formation of carbamoyl phosphate, carbamoyl aspartate, and dihydroorotate. The carbamoyl synthetase activity of this complex is termed carbamoyl phosphate synthetase II (CPS-II) as opposed to CPS-I, which is involved in the urea cycle (Chapter 29). The CPS-II domain of ATCase is activated by ATP and inhibited by UDP, UTP, dUTP, and CTP. As in the regulation of purine synthesis, ATP levels also regulate pyrimidine biosynthesis at the level of PRPP formation. An increase in the level of PRPP results in an activation of pyrimidine synthesis. There is also regulation of OMP decarboxylase: this enzyme is competitively inhibited by UMP and, to a lesser degree, by CMP. Finally, CTP synthase is feedback inhibited by CTP and activated by GTP.
Catabolism of Pyrimidine Nucleotides
Catabolism of the pyrimidine nucleotides leads ultimately to β-alanine (when CMP and UMP are degraded) or β-aminoisobutyrate (when dTMP is degraded) and NH3 and CO2 (Figure 32-9). The β-alanine and β-aminoisobutyrate serve as −NH2 donors in transamination of α-ketoglutarate to glutamate. Subsequent reactions convert the resultant α-ketoacid products to malonyl-CoA, which can be diverted to fatty acid synthesis, or methylmalonyl-CoA, which is converted to succinyl-CoA and can be shunted to the TCA cycle.
FIGURE 32-9: Catabolism of pyrimidines. Hepatic β-ureidopropionase catalyzes the formation of both β-alanine and β-aminoisobutryrate from their pyrimidine precursors. Murray RK, Bender DA, Botham KM, Kennelly PJ, Rodwell VW, Weil PA. Harper’s Illustrated Biochemistry, 29th ed. New York, NY: McGraw-Hill; 2012.
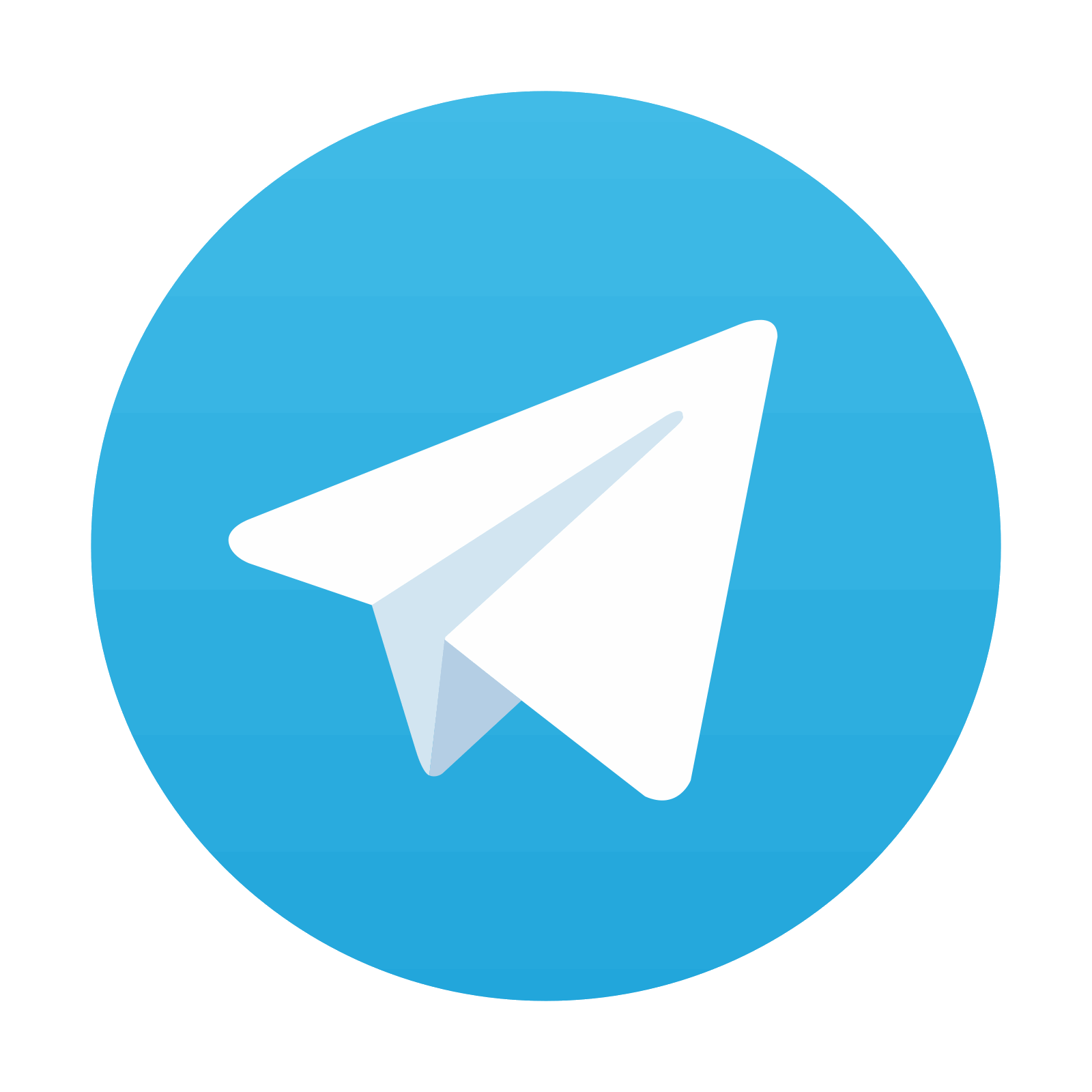
Stay updated, free articles. Join our Telegram channel
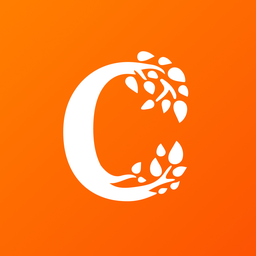
Full access? Get Clinical Tree
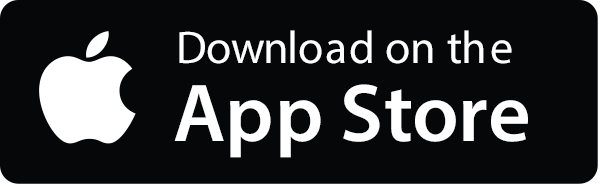
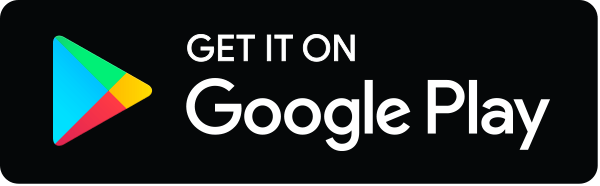