CHAPTER OUTLINE
Cholesterol: Physiologically and Clinically Significant Lipid
Origin of Carbon Atoms in Cholesterol
Reactions of Cholesterol Synthesis
Regulation of Cholesterol Biosynthesis
SREBP and the Regulation of Cellular Sterol Levels
Proteolytic Regulation of HMGR Levels
The Utilization of Cholesterol
Treatment of Hypercholesterolemia
Atorvastatin (Lipitor), Simvastatin (Zocor), Lovastatin (Mevacor)
Gemfibrozil (Lopid), Fenofibrate (TriCor)
Cholestyramine or Colestipol (Resins)
High-Yield Terms
Cholesterol ester: product of fatty acid esterification of the 3-OH catalyzed by ACAT or LCAT
Reverse cholesterol transport: process by which cholesterol is transferred from nonhepatic cells to HDLs and then transported back to the liver via blood
LDL: low-density lipoprotein, derived from de novo VLDL synthesized in the liver, referred to as “bad cholesterol”
HDL: lipoprotein particle involved in reverse cholesterol transport, referred to as “good cholesterol”
SREBP2: sterol-regulated element-binding protein 2, controls the expression of genes involved in hepatic cholesterol biosynthesis
SCAP: SREBP cleavage-activating protein, cleaves the transcription factor domain from ER-embedded SREBP, allowing it to migrate to the nucleus
Insig: insulin-regulated gene, regulates the activity of SCAP
Isoprenoid: any compound containing the 5-carbon organic molecule isoprene (also called terpene) with the formula: CH2=C-(CH3)-CH=CH2
Prenylation: process of attaching isoprenoid intermediates of the cholesterol biosynthetic pathway (farnesyl pyrophosphate and geranylgeranyl pyrophosphate) to proteins
Cholesterol: Physiologically and Clinically Significant Lipid
Cholesterol is a waxy lipophilic compound of the sterol family, which contains 3 six-membered rings, a five-membered ring, and a hydroxyalcohol (Figure 26-1). That cholesterol is a critical component of normal physiological function can easily be demonstrated when its biosynthesis is disrupted or when it accumulates in excess of normal physiological requirements (see Clinical Box 26-1 and 26-2). Loss of the activity of the terminal enzyme in de novo cholesterol biosynthesis (7-dehydrocholesterol reductase [DHCR7]) results in varying degrees of developmental malformation as well as behavioral and learning disabilities encompassing the disorder known as Smith-Lemli-Opitz syndrome (see Clinical Box 26-3). At the opposite end of the spectrum, excess cholesterol, in the vasculature, is a significant contributing factor in the development of coronary artery disease, vascular dysfunction, and atherosclerosis. It is this latter consequence of abnormal cholesterol that has led to the near-universal use of cholesterol biosynthesis–inhibiting drugs in the treatment of hypercholesterolemia.
FIGURE 26-1: Structure of cholesterol.
CLINICAL BOX 26-1: CHOLESTEROL VALUES
Standard fasting blood tests for cholesterol will include values for total cholesterol, HDL cholesterol (so-called “good” cholesterol), and LDL cholesterol (so-called “bad” cholesterol). Family history and lifestyle, including factors such as blood pressure and whether or not one smokes, affect what would be considered ideal versus nonideal values for fasting blood lipid profiles.
Total Serum Cholesterol
<200 mg/dL = desired values
200–239 mg/dL = borderline to high risk
240 mg/dL and above = high risk
HDL Cholesterol
With HDL cholesterol the higher the better
<40 mg/dL for men and <50 mg/dL for women = higher risk
40-50 mg/dL for men and 50-60 mg/dL for women = normal values
>60 mg/dL is associated with some level of protection against heart disease
LDL Cholesterol
With LDL cholesterol the lower the better
<100 mg/dL = optimal values
100 mg/dL-129 mg/dL = optimal to near optimal
130 mg/dL-159 mg/dL = borderline high risk
160 mg/dL-189 mg/dL = high risk
190 mg/dL and higher = very high risk
CLINICAL BOX 26-2: FAMILIAL HYPERCHOLESTEROLEMIA
Familial hypercholesterolemia (FH) (type II hyperlipoproteinemia) is an autosomal dominant disorder that results from mutations affecting the structure and function of the LDL receptor (LDLR). The defects in LDLR interaction with LDL particles result in lifelong elevation of LDL cholesterol in the blood. The consequent hypercholesterolemia leads to premature coronary artery disease and atherosclerotic plaque formation. FH was the first inherited disorder that was recognized as being a cause of myocardial infarction (heart attack).
Although the disease is inherited in an autosomal dominant manner, it exhibits a gene dosage effect. Homozygous individuals are more severely affected than are heterozygotes. Heterozygotes for FH occur with a frequency of 1 in 500, which makes this disease one of the most common inherited disorders in metabolism. More than 700 different mutations in the LDLR gene have been identified. Of diagnostic significance is the fact that 45 different polymorphisms in the LDLR gene have been identified and can be detected using standard molecular biological techniques.
The clinical characteristics of FH include elevated concentrations of plasma LDL and deposition of LDL cholesterol in the arteries, tendons, and skin. Fat deposits in the arteries are called atheromas and in the skin and tendons they are called xanthomas. In heterozygotes, hypercholesterolemia is the earliest clinical manifestation in FH and remains the only clinical finding during the first decade of life. Xanthomas and the characteristic arcus cornea (whitish ring on the peripheral cornea) begin to appear in the second decade. The symptoms of coronary heart disease present in the fourth decade. At the time of death, 80% of heterozygotes will have xanthomas. The clinical picture is more uniform and severe in homozygotes. Homozygotes present with marked hypercholesterolemia at birth and it will persist throughout life. Xanthomas, arcus cornea, and atherosclerosis will develop during childhood in homozygotes. Death from myocardial infarction usually will occur before the age of 30 in homozygotes.
Five different classes of receptor mutation have been identified in FH with each class having multiple alleles. Class 1 mutations are null mutations because these result in a failure to make any detectable LDLR protein. Class 2 mutations are the most common and represent intracellular transport defects. There are 2 subclasses of class 2 mutations in FH. Class 2A mutations result in an LDLR protein that fails to be transported out of the ER. Class 2B mutations are “leaky,” in that some of the newly synthesized LDLR protein is transported to the Golgi but at a reduced rate compared to wild type. The class 2B mutations are the more common type in this class of mutation. Class 3 mutations represent LDLRs that are delivered to the cell surface, but fail in their ability to bind LDL. Because there is a similarity in the class 2 and class 3 mutant alleles, it is difficult to assess which class of mutation is causing the observed FH phenotype. To accurately distinguish class 3 and class 2B alleles at the functional level, it is necessary to isolate fibroblasts from the patient and do in vitro ligand-binding assays. Class 4 mutations are the rarest and result in LDLR protein that will bind LDL, but the LDLR-LDL complexes cannot be internalized. The class 4 alleles can be divided into 2 subclasses dependent upon whether the mutations affect only the cytoplasmic domain or include mutations in the adjacent membrane-spanning domain. Class 5 mutations result in receptors that bind and internalize the LDL particle but cannot release the particles in the endosomes, so the receptors cannot recycle back to the cell surface. Class 5-type mutations may be underestimated because they can produce a phenotype that somewhat resembles that of class 3 mutations (ie, deficient LDL binding).
At the normal end of the spectrum, cholesterol is a critical component of biological membranes, establishing proper fluidity and permeability to the structure. The hydroxyalcohol attached to carbon-3 of the cholesterol structure plays a significant role in the mobilization and storage of cholesterol through fatty acid esterification. In addition to its role in membrane structure and function, cholesterol serves as the precursor for the synthesis of the steroid hormones (see Chapter 50), vitamin D (see Chapter 8), and the bile acids (see Chapter 27). In addition, cholesterol is metabolized by the cytochrome P450 (CYP) family of metabolic enzymes resulting in the generation of a number of oxysterols. Oxysterols serve as biologically active signaling molecules that exert their effects primarily through the activation of nuclear receptors such as the liver X receptors (LXRs, see Chapter 40) and SREBP (see later).
Both dietary cholesterol and that synthesized de novo are transported through the circulation in lipoprotein particles (see Chapter 28) either as free cholesterol or as cholesterol esters with a fatty acid esterified to the carbon-3 hydroxyl group (Figure 26-2). Esterification of cholesterol is catalyzed by ratio of intracellular acyl-CoA to cholesterol acyltransferase (ACAT) or by the ratio of HDL-associated enzyme lecithin to cholesterol acyltransferase (LCAT).
FIGURE 26-2: Fatty acid esterification of cholesterol catalyzed by ACAT (acyl-CoA cholesterol acyltransferase) or LCAT (lecithin cholesterol acyltransferase). Reproduced with permission of themedicalbiochemistrypage, LLC.
Cholesterol Biosynthesis
Critical Enzymes
HMG-CoA reductase (HMGR): rate-limiting enzyme of de novo cholesterol biosynthesis, levels of activity affected at numerous levels
CLINICAL BOX 26-3: SMITH-LEMLI-OPITZ SYNDROME
Smith-Lemli-Opitz syndrome (SLOS) is an autosomal recessive disorder caused by defects in the terminal enzyme of cholesterol biosynthesis: 7-dehydrocholesterol reductase (DHCR7). Defects in this gene result in increased levels of 7-dehydrocholesterol and reduced levels (15%-27% of normal) of cholesterol. The highest frequency of SLOS appears in Caucasians of northern European descent with a frequency of around 1 in 20,000 to 1 in 70,000. The clinical spectrum of SLOS is very broad, ranging from the most severe form manifesting as a lethal malformation syndrome, to a relatively mild disorder that encompasses behavioral and learning disabilities. Frequent observations in SLOS infants is poor feeding and postnatal growth failure. The growth failure may necessitate the insertion of a gastronomy tube to ensure adequate nutritional support. There are distinct craniofacial anomalies associated with SLOS. These include microcephaly (head size smaller than normal), micrognathia (abnormally small lower jaw), ptosis (drooping eyelids), a small upturned nose, and cleft palate or bifid uvula. Male infants with SLOS exhibit genital abnormalities that range from a small penis to ambiguous genitalia or gender reversal. Abnormalities in limb development are common in SLOS patients and include short thumbs, postaxial polydactyly, and single palmar creases. In addition, the most common clinical finding in SLOS patients is syndactyly (fusion of digits) of the second and third toes. This latter limb deformity is found in over 95% of SLOS patients.
The developmental defects are due to defective cholesterol modification of the pattern-regulating gene sonic hedgehog (Shh). Shh is necessary for patterning events that take place in the central nervous system, during limb development, and in the formation of facial structures. Altered levels of cholesterol in SLOS also affect the normal physiochemical processes of cell membranes. One major defect that results from reduced membrane cholesterol is an inability to form ordered lipid domains that are necessary for normal signal transduction events to take place. These defects then alter normal cellular functions resulting in many of the symptoms of SLOS.
7-dehydrocholesterol reductase (DHC7): terminal enzyme of de novo cholesterol biosynthesis; defects lead to defective early development, disease is called SLOS
Normal healthy adults synthesize cholesterol at a rate of approximately 1 g/d and consume approximately 0.3 g/d in the diet. Of course, this assumes a normal healthy diet that does not constitute excess cholesterol intake. A relatively constant level of cholesterol in the blood (150-200 mg/dL) is maintained primarily by controlling the level of de novo synthesis. The synthesis of cholesterol is required only to supply slightly less than half of the daily bodily needs, as the rest is derived from the diet. Biosynthesis in the liver accounts for approximately 10%, and in the intestines approximately 15%, of the amount produced de novo each day.
The rate and level of cholesterol synthesis is regulated by both the dietary intake of cholesterol as well as the cholesterol contributed to the pool by de novo synthesis. Cholesterol from both diet and synthesis is utilized for the synthesis of the bile acids (see Chapter 27) and the steroid hormones (see Chapter 50) as well as being incorporated into cellular membranes. The greatest proportion of cholesterol is consumed in the synthesis of the bile acid in the liver.
Origin of Carbon Atoms in Cholesterol
The synthesis and utilization of cholesterol must be tightly regulated in order to prevent over-accumulation and abnormal deposition within the body. Of particular importance clinically is the abnormal deposition of cholesterol-rich lipoproteins in the coronary arteries. Such deposition, eventually leading to atherosclerosis (see Chapter 48), is the leading contributory factor in diseases of the coronary arteries.
Cholesterol synthesis occurs from the 2-carbon acetate group of acetyl-CoA, which can be derived from glucose oxidation (see Chapter 10), amino acid oxidation (see Chapter 30), and lipid oxidation (see Chapter 25). Since all of these sources of acetyl-CoA originate in the mitochondria and cholesterol synthesis occurs in the cytosol, the acetyl-CoA must be transported out of the mitochondria. Acetyl-CoA transport to the cytoplasm occurs via the mechanism depicted in Figure 19-3.
Acetyl-CoA can also be synthesized from cytosolic acetate derived from cytoplasmic oxidation of ethanol, which is initiated by a cytoplasmic alcohol dehydrogenase (ADH3). The acetyl-CoA synthesis reaction is catalyzed by cytosolic acetyl-CoA synthetase (correctly named acetyl-CoA ligase). Production of acetyl-CoA from ethanol metabolism is one reason that excess alcohol consumption is a significant contributor to elevated serum cholesterol and lipid levels.
Reactions of Cholesterol Synthesis
Cholesterol synthesis is a highly complex process requiring nearly 30 individual reactions that consume over 250 moles of ATP (outlined in Figure 26-3). The second reaction constitutes the rate-limiting reaction, and it is catalyzed by HMG-CoA reductase (HMGR). Cholesterol synthesis can be considered to occur in 5 major steps:
1. Acetyl-CoAs are converted to 3-hydroxy-3-methylglutaryl-CoA (HMG-CoA).
2. HMG-CoA is converted to mevalonate.
3. Mevalonate is converted to the isoprene-based molecule, isopentenyl pyrophosphate (IPP), with the concomitant loss of CO2.
4. IPP is converted to squalene.
5. Squalene is converted to cholesterol.
FIGURE 26-3: Biosynthesis of cholesterol. The numbered positions are those of the steroid nucleus and the open and solid circles indicate the fate of each of the carbons in the acetyl moiety of acetyl-CoA. Murray RK, Bender DA, Botham KM, Kennelly PJ, Rodwell VW, Weil PA. Harper’s Illustrated Biochemistry, 29th ed. New York, NY: McGraw-Hill; 2012.
Each mole of cholesterol begins with the condensation of 3 moles of cytoplasmic acetyl-CoA. First, 2 moles of acetyl-CoA are condensed in a reversal of the thiolase reaction, forming acetoacetyl-CoA. Acetoacetyl-CoA and a third mole of acetyl-CoA are converted to HMG-CoA by the action of cytoplasmic HMG-CoA synthase. This series of reactions is chemically identical to the reactions of ketone body synthesis (see Chapter 25) but of course occur in the cytosol, whereas, the ketone synthesis reactions occur inside the mitochondria. Although the bulk of acetoacetyl-CoA is derived via this process, it is possible for some acetoacetate, generated during ketogenesis to diffuse out of the mitochondria and be converted to acetoacetyl-CoA in the cytosol via the action of acetoacetyl-CoA synthetase.
HMG-CoA is converted to mevalonate by HMG-CoA reductase. HMGR absolutely requires NADPH as a cofactor, and 2 moles of NADPH are consumed during the conversion of HMG-CoA to mevalonate. The reaction catalyzed by HMGR is the rate-limiting step of cholesterol biosynthesis, and this enzyme is subject to complex regulatory controls (see later).
Following the synthesis of mevalonate, the compound is activated by 2 successive phosphorylations (catalyzed by mevalonate kinase and phosphomevalonate kinase), yielding 5-pyrophosphomevalonate. After phosphorylation, an ATP-dependent decarboxylation yields isopentenyl pyrophosphate (IPP), an activated isoprenoid molecule (Figure 26-4). Isopentenyl pyrophosphate is in equilibrium with its isomer, dimethylallyl pyrophosphate (DMPP). One molecule of IPP condenses with one molecule of DMPP to generate geranyl pyrophosphate (GPP). GPP further condenses with another IPP molecule to yield farnesyl pyrophosphate (FPP). Finally, the NADPH-requiring enzyme, squalene synthase, catalyzes the head-to-tail condensation of 2 molecules of FPP, yielding squalene. Like HMGR, squalene synthase is tightly associated with the endoplasmic reticulum. Squalene undergoes a 2-step cyclization to yield lanosterol. The first reaction is catalyzed by squalene monooxygenase. This enzyme uses NADPH as a cofactor to introduce molecular oxygen as an epoxide at the 2,3 position of squalene. Through a series of 19 additional reactions, lanosterol is converted to cholesterol. The terminal reaction in cholesterol biosynthesis is catalyzed by 7-dehydrocholesterol reductase. Defects in this gene results in Smith-Lemli-Opitz syndrome (SLOS, Clinical Box 26-3).
FIGURE 26-4: Biosynthesis of squalene, ubiquinone, dolichol, and other polyisoprene derivatives. (HMG-CoA, 3-hydroxy-3-methylglutaryl-CoA.) A farnesyl residue is present in heme a of cytochrome oxidase. The carbon marked with an asterisk becomes C11 or C12 in squalene. Squalene synthetase is a microsomal enzyme; all other enzymes indicated are soluble cytosolic proteins, and some are found in peroxisomes. Murray RK, Bender DA, Botham KM, Kennelly PJ, Rodwell VW, Weil PA. Harper’s Illustrated Biochemistry, 29th edition, Copyright 2012, New York: McGraw-Hill.
Regulation of Cholesterol Biosynthesis
The cellular supply of cholesterol is maintained at a steady level by 3 distinct mechanisms:
1. Regulation of HMGR activity and levels, which is the major site of control
2. Regulation of excess intracellular free cholesterol through the activity of ratio of acyl-CoA to cholesterol acyltransferase
3. Regulation of plasma cholesterol levels via LDL receptor-mediated uptake and HDL-mediated reverse cholesterol transport
Regulation of HMGR Activity
Regulation of HMGR activity is the primary means for controlling the level of cholesterol biosynthesis. The level of enzyme activity is regulated by 4 distinct mechanisms: (1) feedback inhibition, (2) control of gene expression, (3) rate of enzyme degradation, and (4) phosphorylation-dephosphorylation.
The first 3 control mechanisms are exerted by cholesterol itself. Cholesterol acts as a feedback inhibitor of preexisting HMGR as well as inducing rapid degradation of the enzyme. The latter is the result of cholesterol-induced polyubiquitination of HMGR and its degradation in the proteosome (see Proteolytic Regulation of HMGR Levels section). This ability of cholesterol is a consequence of the sterol-sensing domain (SSD) of HMGR. In addition, when cholesterol is in excess the amount of mRNA for HMGR is reduced because of the decreased expression of the gene. The mechanism by which cholesterol (and other sterols) affects the transcription of the HMGR gene is described in the following section.
Regulation of HMGR through covalent modification occurs because of phosphorylation and dephosphorylation (Figure 26-5). The enzyme is most active in its unmodified form. Phosphorylation of the enzyme decreases its activity. HMGR is phosphorylated by AMP-activated protein kinase (AMPK).
FIGURE 26-5: Regulation of HMGR by covalent modification. HMGR is most active in the dephosphorylated state. Phosphorylation is catalyzed by AMP-activated protein kinase (AMPK) an enzyme whose activity is also regulated by phosphorylation. Phosphorylation of AMPK is catalyzed by at least 2 enzymes: liver kinase B1 (LKB1) and calmodulin-dependent protein kinase kinase-beta (CaMKKβ). Hormones such as glucagon and epinephrine negatively affect cholesterol biosynthesis by increasing the activity of the inhibitor of the phosphatase inhibitor, phosphoprotein phosphatase inhibitor-1, PPI-1. Conversely, insulin stimulates the removal of phosphates and, thereby, activates HMGR activity. Additional regulation of HMGR occurs through an inhibition of its’ activity as well as of its’ synthesis by elevation in intracellular cholesterol levels. Reproduced with permission of themedicalbiochemistrypage, LLC.
The cAMP-signaling pathway via the regulation of PKA activity additionally controls the activity of HMGR. Since the intracellular level of cAMP is regulated by hormonal stimuli, regulation of cholesterol biosynthesis is, therefore, under hormonal control. Insulin decreases cAMP, which in turn activates cholesterol synthesis. Alternatively, glucagon and epinephrine, which increase the level of cAMP, inhibit cholesterol synthesis. In the context of HMGR regulation, PKA phosphorylates phosphoprotein phosphatase inhibitor-1 (PPI-1), leading to an increase in its activity. PPI-1 can inhibit the activity of numerous phosphatases including protein phosphatase-2C (PP-2C) and PP-2A (also known as HMGR phosphatase), which remove phosphates from AMPK and HMGR, respectively. This maintains AMPK in the phosphorylated and active state, and HMGR in the phosphorylated and inactive state. As the stimulus leading to increased cAMP production is removed, the level of phosphorylation decreases and that of dephosphorylation increases. The net result is a return to a higher level of HMGR activity.
SREBP and the Regulation of Cellular Sterol Levels
Long-term control of HMGR activity is exerted primarily through control of the synthesis and degradation of the enzyme. When levels of cholesterol are high, the level of expression of the HMGR gene is reduced. Conversely, reduced levels of cholesterol activate expression of the gene. Insulin also brings about long-term regulation of cholesterol metabolism by increasing the level of HMGR synthesis. The continual alteration of the intracellular sterol pool occurs through the regulation of key sterol-synthetic enzymes as well as by altering the levels of cell-surface LDL receptors. Regulation of the cellular pool of sterols is affected primarily by sterol-regulated transcription of key rate-limiting enzymes and by the regulated degradation of HMGR.
Sterol control over HMGR gene expression occurs through the regulated cleavage of the membrane-bound transcription factor sterol-regulated element-binding protein (SREBP), which binds to sterol-regulatory elements (SREs) in target genes such as the HMGR gene. Both the level and activity of SREBP are regulated by the amount of sterols in the cell (Figure 26-6). There are 2 distinct SREBP genes, SREBP-1 and SREBP-2. In addition, the SREBP-1 gene encodes 2 proteins, SREBP-1a and SREBP-1c. SREBP-2 is the predominant form of this transcription factor in the liver and it exhibits preference for controlling the expression of numerous genes involved in cholesterol homeostasis, including all of the genes encoding the sterol biosynthetic enzymes. In addition, SREBP-2 controls expression of the LDL receptor gene.
FIGURE 26-6: Regulation of SREBP by SCAP: Diagrammatic representation of the interactions between SREBP, SCAP, and Insig in the membrane of the ER when sterols are high. When sterols are low, SCAP does not interact with Insig and the SREBP-SCAP complex migrates to the Golgi where the proteases, S1P and S2P reside. bHLH, basic helix-loop-helix domain; CTD, C-terminal domain; WD, WD40 domain. Reproduced with permission of themedicalbiochemistrypage, LLC.
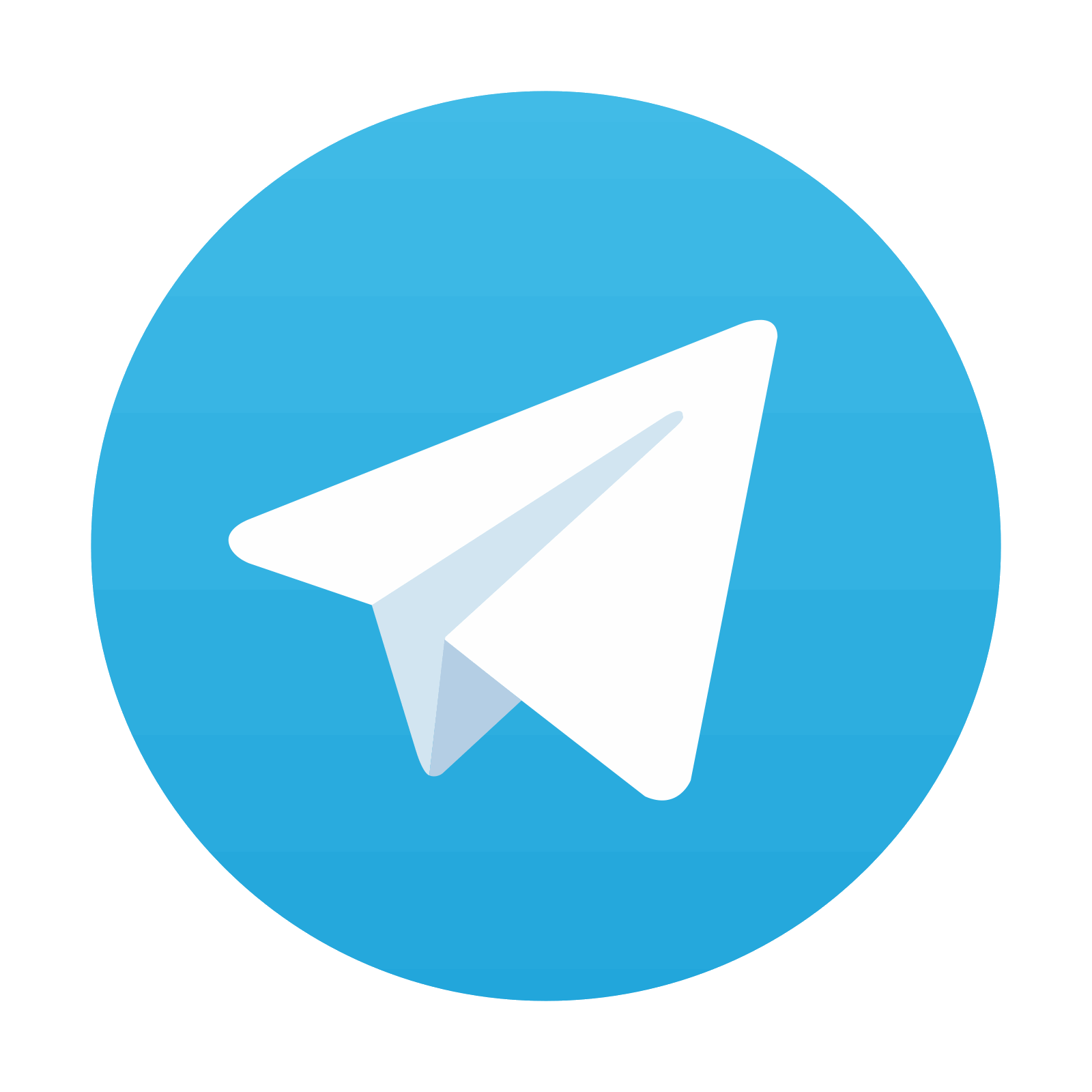
Stay updated, free articles. Join our Telegram channel
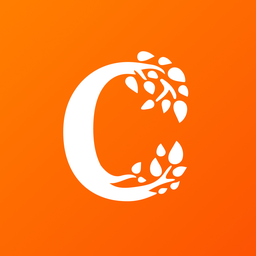
Full access? Get Clinical Tree
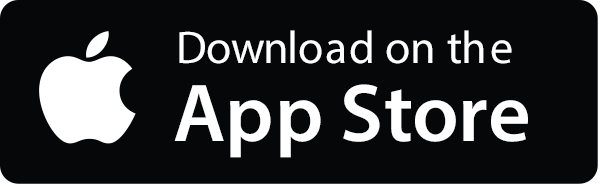
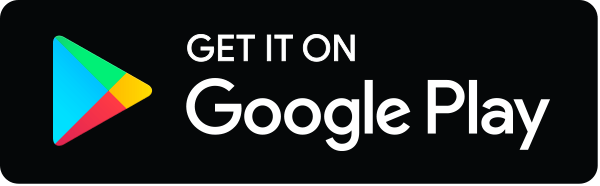