CHAPTER OUTLINE
Lipid Transporters and Cellular Uptake of Fats
Mitochondrial β-Oxidation Reactions
Minor Alternative Fatty Acid Oxidation Pathway
Peroxisomal (alpha) α-Oxidation Pathway
Peroxisomal β-Oxidation Reactions
Microsomal ω-Oxidation Reactions
Regulation of Fatty Acid Metabolism
High-Yield Terms
Adipose tissue triglyceride lipase (ATGL): primary rate-limiting enzyme involved in adipose tissue triglyceride metabolism
Hormone-sensitive lipase (HSL): an adipose tissue–specific hydrolase responsible for the release of fatty acids from stored triglycerides. Its activity is regulated by hormone-mediated phosphorylation
α-oxidation: peroxisomal oxidation pathway responsible for the oxidation of the methyl-substituted fatty acid, phytanic acid present at high concentration in the tissues of ruminants
β-oxidation: major pathway for oxidation of fatty acids in both the mitochondria and the peroxisomes; peroxisomal β-oxidation is also important for metabolism of dicarboxylic acids generated via the ω-oxidation pathway
ω-oxidation: a minor, but clinically significant, pathway for fatty oxidation initiated by microsomal ω-hydroxylation reactions, also involves the oxidation of dicarboxylic acids generated
Ketone body: any of the compounds, β-hydroxybutyrate, acetoacetate, and acetone, generated during hepatic ketogenesis from the substrate, acetyl-CoA
Diabetic ketoacidosis (DKA): a condition of increased plasma ketone body concentration caused by excess adipose tissue fatty acid release and hepatic fatty acid oxidation resulting from unregulated glucagon release
Following absorption of the products of lipid digestion, the resynthesis of TGs, cholesterol esters, and phospholipids occurs. These lipid entities are then solubilized in lipoprotein complexes called chylomicrons. Chylomicrons from the intestine are then released into the blood via the lymph system for delivery to the various tissues for storage or production of energy through oxidation.
Dietary Origins of Lipids
The predominant form of dietary lipid in the human diet is triglyceride (TG or TAG). Gastrointestinal lipid digestion and absorption is discussed in Chapter 43. Briefly, the digestion of dietary triglyceride begins in the stomach with the action of gastric lipase and continues in the duodenum via the concerted actions of gastric lipase and pancreatic lipase. Digestion of dietary phospholipids takes place in the duodenum as well via the action of pancreatic phospholipase A2 (PLA2), yielding free fatty acids and lysophospholipids. Digestion of dietary cholesterol esters results via the action of carboxyl ester lipase (CEL). Intestinal absorption of diacyl- and monoacylglycerides, lysophospholipids, free fatty acids, and cholesterol occurs at the interface between the brush border membranes of intestinal enterocytes and the lipid micelles and involves both passive diffusion and transport protein–mediated uptake mechanisms.
Discussion of the various lipoproteins present in human circulation can be found in Chapter 28. As chylomicrons circulate in the vasculature, fatty acids are removed from the TG fraction through the action of endothelial cell–associated lipoprotein lipase (LPL). The free fatty acids are then absorbed by the cells and the glycerol is returned via the blood to the liver where it is utilized as a carbon skeleton for glucose synthesis via gluconeogenesis (Chapter 13).
Mobilization of Fat Stores
The primary sources of fatty acids for oxidation are dietary and mobilization from cellular stores. Fatty acids from the diet are absorbed from the gut, packaged into lipoprotein particles called chylomicrons within intestinal enterocytes and then delivered to cells of the body via transport in the blood. Fatty acids are stored in the form of triglycerides (TAGs or TGs) within all cells but predominantly within adipose tissue. In response to energy demands, the fatty acids of stored TGs can be mobilized for use by peripheral tissues. The release of these stored fatty acids is controlled by a complex series of interrelated cascades that result in the activation of triglyceride hydrolysis. The primary intracellular lipases involved in the mobilization of stored fatty acids are adipose triglyceride lipase (ATGL, also called desnutrin), hormone-sensitive lipase (HSL), and monoacylglyceride lipase (MGL).
Adipose Triglyceride Lipase
Adipose triglyceride lipase (ATGL)/desnutrin belongs to the family of patatin domain-containing proteins that consists of 9 human members. Because some members of the family act as phospholipases, the proteins were originally called patatin-like phospholipase domain-containing proteins A1 to A9 (PNPLA1–PNPLA9). ATGL (designated PNPLA2 in the patatin domain nomenclature) preferentially hydrolyzes TGs. ATGL expression and enzyme activity are both under complex regulation. Expression of ATGL is induced by peroxisome proliferator–activated receptor (PPAR) agonists, glucocorticoids, and fasting. The level of lipase activity of ATGL (as well as HSL) does not always correlate to the level of expression of the gene. The discrepancy between ATGL and HSL mRNA level and the level of enzyme activity is the result of extensive posttranslational regulation of both of these lipases.
High-Yield Concept
An additional lipase, lysosomal acid lipase (LAL), plays an important role in the intracellular degradation of cholesterol esters and TGs taken into cells via endocytosis and transferred to the lysosomes. The importance of LAL in overall lipid homeostasis is evidenced by the fact that LAL deficiency results in the significant accumulation of cholesterol esters in tissues such as the spleen and liver. LAL deficiency is commonly called Wolman disease.
Of clinical significance is the recent observation that blocking perilipin-2 (Plin2) activity in mice leads to inhibition of diet-induced obesity. This response is associated with increased formation of subcutaneous beige adipocytes that express UCP1, a reduction of inflammatory foci formation in white adipose tissue (WAT) as well as reduced steatosis in the liver. Loss of Plin2 activity results in reduced energy intake and increased physical activity in response to a high-fat diet indicating that Plin2 likely contributes to diet-induced obesity, adipose tissue inflammation, and the development of hepatic steatosis.
There are 2 known serine residues in ATGL that are subject to phosphorylation. Unlike phosphorylation of HSL (described below), ATGL phosphorylation is not PKA-dependent. Some lines of evidence suggest that AMPK phosphorylates ATGL resulting in increased lipase activity. In addition to phosphorylation regulating ATGL activity, the enzyme requires a coactivator protein for full activity. This coactivator is known as comparative gene identification-58 (CGI-58). The official nomenclature for CGI-58 is α/β-hydrolase domain-containing protein-5 (ABHD5), owing to the presence of an α/β-hydrolase domain commonly found in esterases, thioesterases, and lipases. Additional proteins that are associated with lipid droplets (LD) in adipocytes participate in the ABHD5-mediated regulation of ATGL such as perilipin-1, Plin1.
In nonadipose tissues with high rates of TG hydrolysis, such as skeletal muscle and liver, regulation of ATGL activity occurs via a mechanism distinct from that in adipose tissues. In these tissues, perilipin-1 is replaced by perilipin-5 which recruits both ATGL and ABHD5 to LD by direct binding of the enzyme and its coactivator. Other perilipins exist in cells including perilipin-2, -3, and -4 but it is unclear if these proteins are also involved in regulating the association of ATGL with LD.
Hormone-sensitive Lipase
The expression profile of hormone-sensitive lipase (HSL) essentially mirrors that of ATGL. Highest mRNA and protein concentrations are found in WAT and brown adipose tissue (BAT) with low levels of expression found in muscle, testis, steroidogenic tissues, and pancreatic islets as well as several other tissues. HSL was originally thought to be rate limiting for the catabolism of fat stores in adipose tissue and many nonadipose tissues. However, HSL actually has a higher level of activity as a diglyceride (DG) hydrolase than as a TG hydrolase. This became evident when HSL-deficient mice were produced and shown to efficiently hydrolyze TG and they do not accumulate TG in either adipose or nonadipose tissues, but they do accumulate large amounts of DGs in many tissues. It is now accepted that ATGL is responsible for the initial step of lipolysis in human adipocytes, and that HSL is rate-limiting for the catabolism of DG. HSL not only hydrolyzes DG but is also active at hydrolyzing ester bonds of many other lipids including TG, MG, cholesteryl esters, retinyl esters, and short-chain carbonic acid esters.
In adipose tissue, HSL activity is strongly induced by β-adrenergic stimulation; conversely insulin has a strong inhibitory effect. While β-adrenergic stimulation regulates ATGL primarily via recruitment of the coactivator ABHD5, HSL is a major target for PKA-mediated phosphorylation. Additional kinases, including AMPK, extracellular signal-regulated kinase (ERK), glycogen synthase kinase-4 (GSK-4), and Ca2+/calmodulin-dependent kinase 1 (CAMK1), also phosphorylate HSL to modulate the activity of the enzyme. Although phosphorylation affects HSL activity, full activation requires access to LD, which in adipose tissue is mediated by Plin1 (Figure 25-1).
FIGURE 25-1: Control of adipose tissue lipolysis. (FFA, free fatty acids; TSH, thyroid-stimulating hormone.) Note the cascade sequence of reactions affording amplification at each step. The lipolytic stimulus is “switched off” by removal of the stimulating hormone; the action of lipase phosphatase; the inhibition of the lipase and adenylyl cyclase by high concentrations of FFA; the inhibition of adenylyl cyclase by adenosine; and the removal of cAMP by the action of phosphodiesterase. ACTH, TSH, and glucagon may not activate adenylyl cyclase in vivo since the concentration of each hormone required in vitro is much higher than is found in the circulation. Positive () and negative
regulatory effects are represented by broken lines and substrate flow by solid lines. Murray RK, Bender DA, Botham KM, Kennelly PJ, Rodwell VW, Weil PA. Harper’s Illustrated Biochemistry, 29th ed. New York, NY McGraw-Hill; 2012.
In nonadipose tissues, such as skeletal muscle, HSL is activated by phosphorylation in response to epinephrine (β-adrenergic receptor–mediated activation of PKA) and muscle contraction (calcium release from sarcoplasmic reticulum).
Insulin-mediated deactivation of lipolysis is associated with transcriptional downregulation of ATGL and HSL expression. Insulin signaling also results in the activation of various phosphodiesterase (PDE) isoforms leading to PDE-catalyzed hydrolysis of cAMP which in turn results in reduced activation of PKA. These actions turn off lipolysis by preventing phosphorylation and activation of HSL. In addition to its peripheral action, insulin also functions within the sympathetic nervous system to inhibit lipolysis in WAT. Increased insulin levels in the brain inhibit both HSL and Plin1 phosphorylation which results in reduced HSL and ATGL activities.
Monoacylglyceride Lipase
Monoacylglyceride lipase (MGL) is considered to be the rate-limiting enzyme for the breakdown of MG that are the result of both extracellular and intracellular lipolysis pathways. The extracellular generation of MG is the result of the action of endothelial cell lipoprotein lipase (LPL) on lipoprotein particle–associated TG. Intracellular hydrolysis of TG by ATGL and HSL as well as intracellular phospholipid hydrolysis by phospholipase C (PLC) and membrane-associated DG lipase α and β results in the generation of MGL substrates.
MGL is ubiquitously expressed with highest levels of expression in adipose tissue. MGL shares homology with esterases, lysophospholipases, and haloperoxidases. MGL is critically important for efficient degradation of MG since it has been shown in mouse models that lack of MGL impairs lipolysis and is associated with increased MG levels in adipose and nonadipose tissues alike. MGL has received particular attention in recent years due to the discovery that the enzyme is responsible for the inactivation of 2-arachidonoylglycerol (2-AG) which is an endogenous cannabinoid (endocannabinoid).
Lipid Transporters and Cellular Uptake of Fats
When fatty acids are released from adipose tissue stores, they enter the circulation as free fatty acids (FFA) and are bound to albumin for transport to peripheral tissues. When the fatty acid-albumin complexes interact with cell surfaces, the dissociation of the fatty acid from albumin represents the first step of the cellular uptake process. Cellular uptake of fatty acids involves several members of the fatty acid receptor family including fatty acid translocase (FAT/CD36), plasma membrane-associated fatty acid-binding protein (FABPpm), and at least 6 fatty acid transport proteins (FATP1-FATP6). The FATP facilitate the uptake of very-long-chain (VLCFA) and long-chain fatty acids (LCFA) (Table 25-1).
Following uptake into cells, fatty acids must be activated to acyl-CoA intermediates. Several members of the FATP family possess acyl-CoA synthetase (ACS) activity. The overall process of cellular fatty acid uptake and subsequent intracellular utilization represents a continuum of dissociation from albumin by interaction with the membrane-associated transport proteins, activation to acyl-CoA (in many cases via FATP action) followed by intracellular trafficking to sites of metabolic disposition.
Mitochondrial β-Oxidation Reactions
The primary sites of fatty acid β-oxidation are the mitochondria and the peroxisomes. Fatty acids of between 4 and 8 and between 6 and 12 carbon atoms in length, referred to as short- and medium-chain fatty acids (SCFA and MCFA), respectively, are oxidized exclusively in the mitochondria. Long-chain fatty acids (LCFA: 10-16 carbons long) are oxidized in both the mitochondria and the peroxisomes with the peroxisomes exhibiting preference for 14-carbon and longer LCFA. Very-long-chain fatty acids (VLCFA: 17-26 carbons long) are preferentially oxidized in the peroxisomes.
Fatty acids must be activated in the cytoplasm before being oxidized in the mitochondria. Activation is catalyzed by fatty acyl-CoA synthetases (also called acyl-CoA ligases or thiokinases). The net result of this activation process is the consumption of 2 molar equivalents of ATP. The process of mitochondrial fatty acid oxidation is termed β-oxidation since it occurs through the sequential removal of 2-carbon units by oxidation at the β-carbon position of the fatty acyl-CoA molecule.
The transport of fatty acyl-CoA into the mitochondria is accomplished via an acyl-carnitine intermediate, which itself is generated by the action of carnitine palmitoyltransferase 1 (CPT-1 or CPT-I) an enzyme that resides in the outer mitochondrial membrane (Figure 25-2 and Clinical Box 25-1). There are 3 CPT-1 genes in humans identified as CPT-1A, CPT-1B, and CPT-1C. Expression of CPT-1A predominates in the liver and is thus, referred to as the liver isoform. CPT-1B expression predominates in skeletal muscle and is thus, referred to as the muscle isoform. CPT-1C expression is exclusive to the brain and testes. The activity of CPT-1C is distinct from those of CPT-1A and CPT-1B in that it does not act on the same types of fatty acyl-CoAs that are substrates for the latter 2 enzymes. However, CPT-1C does exhibit high-affinity malonyl-CoA binding. Following carnitine acyl-carnitine–mediated transfer of the CPT-1-generated fatty acyl-carnitines across the inner mitochondrial membrane, the fatty acyl-carnitine molecules are acted on by the inner mitochondria membrane carnitine palmitoyltransferase 2 (CPT-2 or CPT-II) regenerating the fatty acyl-CoA molecules (Figure 25-2).
FIGURE 25-2: Role of carnitine in the transport of long-chain fatty acids through the inner mitochondrial membrane. Long-chain acyl-CoA cannot pass through the inner mitochondrial membrane, but its metabolic product, acylcarnitine, can. Murray RK, Bender DA, Botham KM, Kennelly PJ, Rodwell VW, Weil PA. Harper’s Illustrated Biochemistry, 29th ed. New York, NY McGraw-Hill; 2012.
Each round of β-oxidation involves 4 steps that, in order, are oxidation, hydration, oxidation, and cleavage (Figure 25-3, steps 2-5). The first oxidation step β-oxidation involves a family of FAD-dependent acyl-CoA dehydrogenases. Each of these dehydrogenases has a range of substrate specificity determined by the length of the fatty acid. Short-chain acyl-CoA dehydrogenase (SCAD, also called butyryl-CoA dehydrogenase) prefers fats of 4 to 6 carbons in length; medium-chain acyl-CoA dehydrogenase (MCAD; see Clinical Box 25-2) prefers fats of 4 to 16 carbons in length with maximal activity for C10 acyl-CoAs; long-chain acyl-CoA dehydrogenase (LCAD) prefers fats of 6 to 16 carbons in length with maximal activity for C12 acyl-CoAs. Deficiencies in several of the acyl-CoA dehydrogenases are known to result in impaired β-oxidation with MCAD deficiency being the most common form.
FIGURE 25-3: β-Oxidation of fatty acids. Long-chain acyl-CoA is cycled through reactions 2-5, acetyl-CoA being split off, each cycle, by thiolase (reaction 5). When the acyl radical is only 4 carbon atoms in length, 2 acetyl-CoA molecules are formed in reaction 5. Murray RK, Bender DA, Botham KM, Kennelly PJ, Rodwell VW, Weil PA. Harper’s Illustrated Biochemistry, 29th ed. New York, NY McGraw-Hill; 2012.
The next 3 steps in mitochondrial β-oxidation involve a hydration step, another oxidation step, and finally a hydrolytic reaction that requires CoA and releases acetyl-CoA and an acyl-CoA 2 carbon atoms shorter than the initial substrate. The water addition is catalyzed by an enoyl-CoA hydratase activity, the second oxidation step is catalyzed by an NAD-dependent long-chain hydroxyacyl-CoA dehydrogenase activity (3-hydroxyacyl-CoA dehydrogenase activity), and finally the cleavage into an acyl-CoA and an acetyl-CoA is catalyzed by a thiolase activity. These 3 activities are encoded in a multifunctional enzyme called the mitochondrial trifunctional protein (MTP). MTP is composed of 8 protein subunits, 4 α-subunits encoded by the HADHA gene, and 4 β-subunits encoded by the HADHB gene. The α-subunits contain the enoyl-CoA hydratase and long-chain hydroxyacyl-CoA dehydrogenase activities, while the β-subunits possess the 3-ketoacyl-CoA thiolase (β-ketothiolase or just thiolase) activity. The mammalian genome actually encodes 5 distinct enzymes with thiolase activity (Table 25-2).
Each round of β-oxidation produces 1 mole of FADH2, 1 mole of NADH, and 1 mole of acetyl-CoA. The acetyl-CoA, the end product of each round of β-oxidation, then enters the TCA cycle (Chapter 13), where it is further oxidized to CO2 with the concomitant generation of 3 moles of NADH, 1 mole of FADH2 and 1 mole of ATP. The NADH and FADH2 generated during the fat oxidation and acetyl-CoA oxidation in the TCA cycle then can enter the respiratory pathway for the production of ATP via oxidative phosphorylation (Chapter 14).
Minor Alternative Fatty Acid Oxidation Pathway
The majority of natural lipids contain an even number of carbon atoms. A small proportion of plant-derived lipids contain odd numbers and upon complete β-oxidation these yield acetyl-CoA units plus a single mole of propionyl-CoA. The propionyl-CoA is converted in an ATP-dependent pathway to succinyl-CoA. The succinyl-CoA can then enter the TCA cycle for further oxidation (Figure 25-4).
FIGURE 25-4: Conversion of propionyl-CoA to succinyl-CoA. Reproduced with permission of themedicalbiochemistrypage, LLC.
CLINICAL BOX 25-1: CARNITINE-RELATED ABNORMALITIES
Deficiencies in carnitine and the activity of the carnitine palmitoyltransferases lead to impaired energy production from fatty acid oxidation. Deficiencies in carnitine lead to an inability to transport fatty acids into the mitochondria for oxidation. This can occur in newborns and particularly in preterm infants. Carnitine deficiencies also are found in patients undergoing hemodialysis or exhibiting organic aciduria. Carnitine deficiencies may manifest systemic symptomology or may be limited to only muscles. Symptoms can range from mild occasional muscle cramping to severe weakness or even death. Treatment is by oral carnitine administration. Deficiencies in CPT-I are relatively rare and affect primarily the liver and lead to reduced fatty acid oxidation and ketogenesis. The most common symptom associated with CPT-I deficiency is hypoketotic hypoglycemia. There is also an elevation in blood levels of carnitine. The liver involvement results in hepatomegaly and in muscles results in weakness. CPT-II deficiencies can be classified into 3 main forms. The adult form affects primarily the skeletal muscles and is called the adult myopathic form. This form of the disease causes muscle pain and fatigue and myoglobinuria following exercise. The severe infantile multisystem form manifest in the first 6 to 24 months of life with most afflicted infants demonstrating significant involvement before 1 year. The primary symptom of this form of CPT-II deficiency is hypoketotic hypoglycemia. Symptoms will progress to severe hepatomegaly and cardiomyopathy. Often death from CPT-II deficiency may be misdiagnosed as sudden infant death syndrome (SIDS). The rarest form of CPT-II deficiency is referred to as the neonatal lethal form. Symptoms of this form appear within hours to 4 days after birth and include respiratory failure, hepatomegaly, seizures, hypoglycemia, and cardiomegaly. The cardiomegaly will lead to fatal arrhythmias. Carnitine acyltransferases may also be inhibited by sulfonylurea drugs such as tolbutamide and glyburide.
CLINICAL BOX 25-2: MEDIUM-CHAIN ACYL-COA DEHYDROGENASE DEFICIENCY
Medium-chain acyl-CoA dehydrogenase (MCAD), which is also called acyl-CoA dehydrogenase, medium-chain (ACADM), is so called because of the size range of its fatty acyl-CoA substrates. MCAD acts on fatty acyl-CoA molecules that range in size from 12 down to 4 carbons in length. Deficiency in MCAD is the most common defect observed in the process of mitochondrial β-oxidation of fatty acids. In fact, MCAD deficiency is one of the most common inherited disorders of metabolism occurring with a frequency of approximately 1 in 10,000 live births. The most common symptom of MCAD deficiency is episodic hypoketotic hypoglycemia brought on by fasting. Symptoms appear within the first 2 years of life. Clinical crisis is characterized by an infant presenting with episodes of vomiting and lethargy that may progress to seizures and ultimately coma. A prior upper respiratory or gastrointestinal viral infection will lead to reduced oral intake in these infants which can precipitate the acute crisis. The first episode in an infant may be fatal and the death ascribed to sudden infant death syndrome (SIDS). Autopsy results will often find marked fatty liver (hepatic steatosis) and cerebral edema. These findings are sometimes misdiagnosed as Reye syndrome especially in the circumstance where there has been a prior infection. Because the capacity of the gluconeogenesis pathway is limited in newborn infants, they are highly susceptible to the lack of brain energy from the ketones that would normally be derived from fatty acid oxidation. The defect in fatty acid oxidation leads to the presence, in the plasma and urine, of toxic metabolic intermediates such as dicarboxylic acids. Characteristic of, and diagnostic for, MCAD deficiency is the presence of octanoylcarnitine. Diagnosis can be made within 24 to 48 hours in specialized laboratories using tandem mass spectrometry (MS) of the blood for specific acylcarnitines. In MCAD deficiency the acylcarnitines that are found are highly diagnostic and specific for this disorder and include C6:0-, 4-cis-, and 5-cis-C8:1, C8:0, and 4-cis-C10:1 acylcarnitine species. The primary goal of treatment for MCAD deficiency patients is to provide adequate caloric intake, the avoidance of fasting and IV glucose to treat acute episodes, along with aggressive therapy during periods of infection. Anorexia can develop during infections and fever leading to mobilization of stored fatty acids. The effect of increased lipid mobilization is the production of toxic intermediates which can lead to vomiting, lethargy, coma, and even death. Treatment with oral carnitine increases the removal of these toxic intermediates.
The oxidation of unsaturated fatty acids is essentially the same process as for saturated fats, except when a double bond is encountered (Figure 25-5). In such a case, the bond is isomerized by a specific enoyl-CoA isomerase and oxidation continues. In the case of linoleate, the presence of the Δ12 unsaturation results in the formation of a dienoyl-CoA during oxidation. This molecule is the substrate for an additional oxidizing enzyme, the NADPH requiring 2,4-dienoyl-CoA reductase.
FIGURE 25-5: Sequence of reactions in the oxidation of unsaturated fatty acids, for example, linoleic acid. Δ4–cis-fatty acids or fatty acids forming Δ4–cis-enoyl-CoA enter the pathway at the position shown. NADPH for the dienoyl-CoA reductase step is supplied by intramitochondrial sources such as glutamate dehydrogenase, isocitrate dehydrogenase, and NAD(P)H transhydrogenase. Murray RK, Bender DA, Botham KM, Kennelly PJ, Rodwell VW, Weil PA. Harper’s Illustrated Biochemistry, 29th ed. New York, NY McGraw-Hill; 2012.
Peroxisomal (Alpha) α-Oxidation Pathway
Phytanic acid is a fatty acid present in the tissues of ruminants and in dairy products and is, therefore, an important dietary component of fatty acid intake. Because phytanic acid is methylated, it cannot act as a substrate for the normal mitochondrial β-oxidation pathway. Phytanic acid is first converted to its CoA-ester and then phytanoyl-CoA serves as a substrate in an α-oxidation process, therefore, the process is referred to as α-oxidation (Figure 25-6). The α-oxidation reaction, as well as the remainder of the reactions of phytanic acid oxidation, occurs within the peroxisomes and requires a specific α-hydroxylase called phytanoyl-CoA hydroxylase (PhyH). The action of PhyH adds a hydroxyl group to the α-carbon of phytanic acid generating the 19-carbon homologue, pristanic acid. Pristanic acid then serves as a substrate for the remainder of the normal process of β-oxidation. Deficiency in PhyH activity results in the disorder known as Refsum disease. (see Clinical Box 25-3).
FIGURE 25-6: α-oxidation reactions involved the metabolism of phytanic acid. Reproduced with permission of themedicalbiochemistrypage, LLC.
Peroxisomal β-Oxidation Reactions
In addition to mitochondrial oxidation of fatty acids, the peroxisomes play an important role in overall fatty acid metabolism. Very-long-chain fatty acids (VLCFAs: 17-26 carbons long) are preferentially oxidized in the peroxisomes with cerotic acid (a 26:0 fatty acid) being solely oxidized in this organelle. The peroxisomes also metabolize di- and trihydroxycholestanoic acids (bile acid intermediates); long-chain dicarboxylic acids that are produced by ω-oxidation of long-chain monocarboxylic acids (see below); pristanic acid via the α-oxidation pathway (see above); certain polyunsaturated fatty acids (PUFA) such as tetracosahexaenoic acid (24:6), which by β-oxidation yields the important PUFA docosahexaenoic acid (DHA); and certain prostaglandins and leukotrienes.
The enzymatic processes of peroxisomal β- oxidation are very similar to those of mitochondrial β-oxidation with 1 major difference (Figure 25-7). During mitochondrial oxidation the first oxidation step, catalyzed by various acyl-CoA dehydrogenases, results in the reduced electron carrier FADH2 that then delivers its electrons directly to the electron transport chain for synthesis of ATP. In the peroxisome the first oxidation step is catalyzed by acyl-CoA oxidases which are coupled to the reduction of O2 to hydrogen peroxide (H2O2). Thus, the reaction is not coupled to energy production but instead yields a significant reactive oxygen species (ROS). Peroxisomes contain the enzyme catalase that degrades the hydrogen peroxide back to O2.
FIGURE 25-7: Pathway of peroxisomal β-oxidation. Fatty acids are taken into the peroxisome and esterified to CoA by the solute carrier family member proteins ABCD1, ABCD2, or ABCD3. ABCD1 is also called VLCFA-CoA synthetase. DBP is D-bifunctional protein. The peroxisomal thiolase indicated in the figure is ACAA1 (acetyl-CoA C-acyltransferase 1, also known as peroxisomal 3-oxoacyl-CoA thiolase). Acetyl-CoA generated by peroxisomal β-oxidation is transported out of the peroxisome after exchange of carnitine for the CoA. Mitochondrial acetyl-carnitine is formed through the action of carnitine acetyltransferase (CAT). Acetyl-carnitine is transported out of the mitochondria via the action of carnitine-acylcarnitine translocase (CACT). Once in the cytosol acetyl-carnitine is converted to acetyl-CoA via the action of cytosolic CAT. These acetyl-CoA units can be used for cytosolic fatty acid synthesis or imported into the mitochondria for oxidation in the TCA cycle. Reproduced with permission of themedicalbiochemistrypage, LLC.
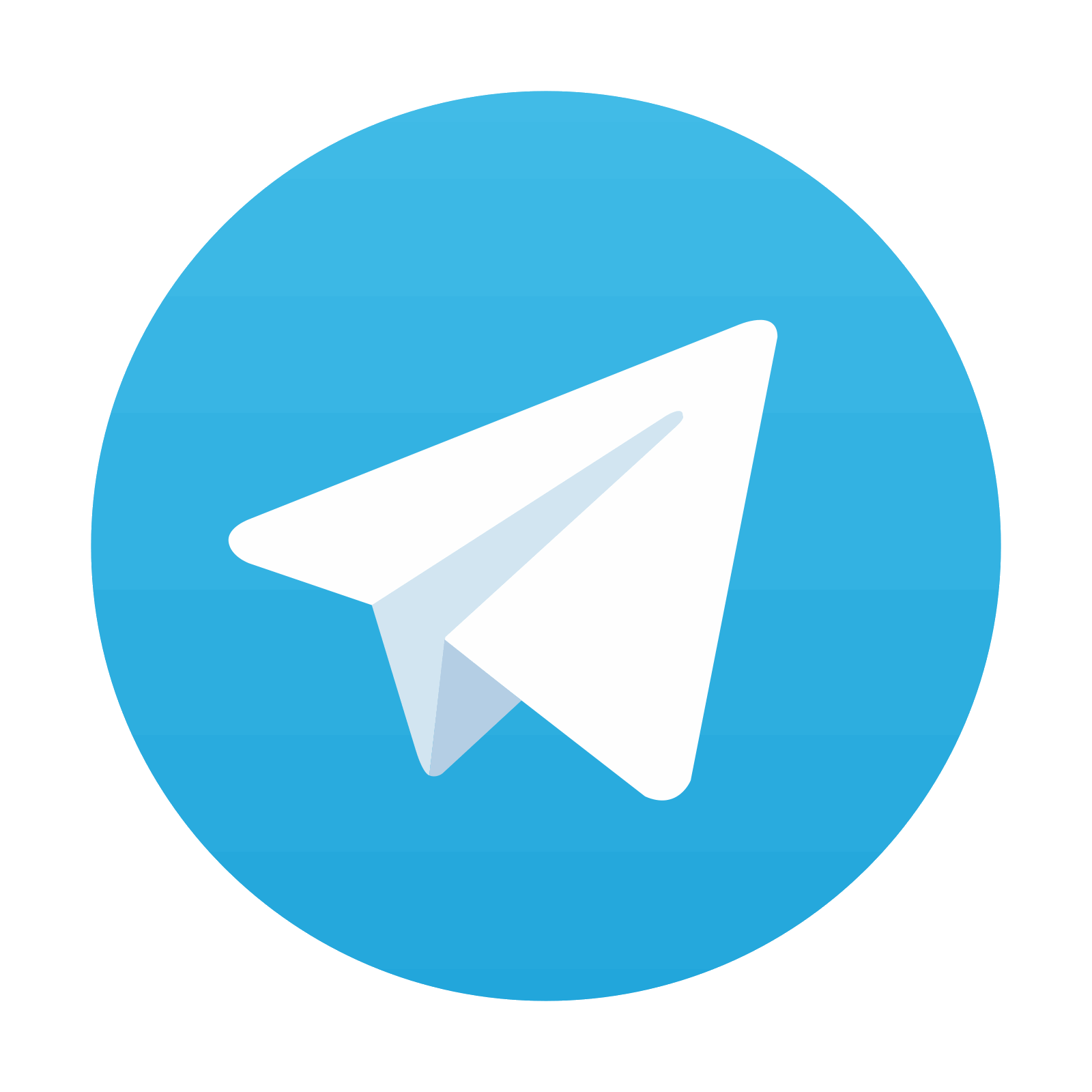
Stay updated, free articles. Join our Telegram channel
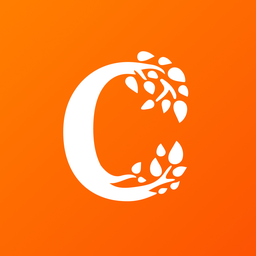
Full access? Get Clinical Tree
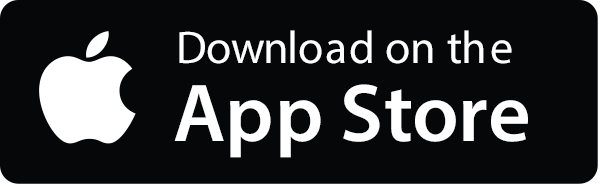
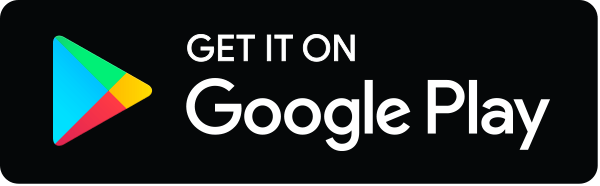