CHAPTER OUTLINE
Origin of Cytoplasmic Acetyl-CoA
ATP-Citrate Lyase: Role in Epigenetics
Regulation of Fatty Acid Synthesis
ChREBP: Master Lipid Regulator in the Liver
Synthesis of Biologically Active Omega-3 and Omega-6 Polyunsaturated Fatty Acids
High-Yield Terms
ATP-citrate lyase (ACL): cytosolic enzyme involved in the transport of acetyl-CoA from the mitochondria to the cytosol; a nuclear ACL is involved in delivery of acetyl-CoA to histone acetyltransferases (HAT) involved in modulating gene expression at the level of epigenetic marking
Acetyl-CoA carboxylase (ACC): rate-limiting and highly regulated enzyme of de novo fatty acid synthesis
Fatty acid synthase (FAS): homodimeric enzyme that carries out all of the reactions of de novo fatty acid synthesis generating palmitic acid as the final product
Carbohydrate-response element-binding protein: major glucose-responsive transcription factor that is required for glucose-induced expression of L-PK and the lipogenic genes ACC and FAS
Steroyl-CoA desaturase (SCD): rate-limiting enzyme for the synthesis of monounsaturated fatty acids (MUFAs), primarily oleate (18:1) and palmitoleate (16:1), both of which represent the majority of MUFA present in membrane phospholipids, triglycerides, and cholesterol esters
De Novo Fatty Acid Synthesis
The pathway for fatty acid synthesis occurs in the cytoplasm, utilizes the oxidation of NADPH, and requires an activated intermediate. The activated intermediate is malonyl-CoA which is derived via carboxylation of acetyl-CoA. The synthesis of malonyl-CoA represents the first committed step as well as the rate-limiting and major regulated step of fatty acid synthesis (Figure 19-1). This reaction is catalyzed by the biotin-requiring enzyme, acetyl-CoA carboxylase (ACC). Human tissues express 2 distinct ACC genes identified as ACC1 and ACC2. The differential regulation of these 2 genes and the encoded enzymes is discussed in the Regulation of Fatty Acid Synthesis section.
FIGURE 19-1: Synthesis of malonyl-CoA catalyzed by acetyl-CoA carboxylase. Reproduced with permission of themedicalbiochemistrypage, LLC.
The reactions of fatty acid synthesis are catalyzed by fatty acid synthase (FAS) (Figure 19-2). All of the reactions of fatty acid synthesis take place in distinct reactive centers within the enzyme. These reactive centers are β-keto-ACP synthase, β-keto-ACP reductase, 3-OH acyl-ACP dehydratase and enoyl-CoA reductase. ACP is acyl-carrier protein but does not refer to a distinct protein but rather refers to a distinct domain of the enzyme which contains a phosphopantetheine group. The 2 reduction reactions require NADPH oxidation to NADP+. The acetyl-CoA and malonyl-CoA are transferred to ACP by the action of acetyl-CoA transacylase and malonyl-CoA transacylase, respectively. The attachment of these carbon atoms to ACP allows them to enter the fatty acid synthesis cycle. The primary fatty acid synthesized by FAS is palmitate. Palmitate is then released from the enzyme and can then undergo separate elongation and/or unsaturation to yield other fatty acid molecules.
FIGURE 19-2: Reactions of fatty acid synthesis catalyzed by FAS. Only half of the normal head-to-tail (head-to-foot) dimer of functional FAS is shown. Synthesis of malonyl-CoA from CO2 and acetyl-CoA is carried out by ACC as shown in Figure 19-1. The acetyl group is initially attached to the sulfhydryl of the 4′-phosphopantothenate of the acyl carrier protein portion of FAS (ACP-SH). This is catalyzed by malonyl/acetyl-CoA ACP transacetylase (reactions 1 and 2). This activating acetyl group represents the omega (ω) end of the newly synthesized fatty acid. Following transfer of the activating acetyl group to a cysteine sulfhydryl in the β-keto-ACP synthase portion of FAS (CYS-SH), the three carbons from a malonyl-CoA are attached to ACP-SH (reaction 3), also catalyzed by malonyl/acetyl-CoA ACP transacetylase. The acetyl group attacks the methylene group of the malonyl attached to ACP-SH catalyzed β-keto-ACP synthase (reaction 4) which also liberates the CO2 that was added to acetyl-CoA by ACC. The resulting 3-ketoacyl group then undergoes a series of 3 reactions catalyzed by the β-keto-ACP reductase (reaction 5), 3-OH acyl-ACP dehydratase (reaction 6), and enoyl-CoA reductase (reaction 7) activities of FAS resulting in a saturated 4-carbon (butyryl) group attached to the ACP-SH. This butyryl group is then transferred to the CYS-SH (reaction 8) as for the case of the activating acetyl group. At this point another malonyl group is attached to the ACP-SH (3b) and the process begins again. Reactions 4 through 8 are repeated another 6 times, each beginning with a new malonyl group being added. At the completion of synthesis, the saturated 16-carbon fatty acid, palmitic acid, is released via the action of the thioesterase activity of FAS (palmitoyl ACP thioesterase) located in the C-terminal end of the enzyme. Not shown are the released CoASH groups. Reproduced with permission of themedicalbiochemistrypage, LLC.
Origin of Cytoplasmic Acetyl-CoA
Acetyl-CoA is generated in the mitochondria primarily from 2 sources, the pyruvate dehydrogenase complex (PDHc) reaction and fatty acid oxidation but also can originate from the oxidation of several amino acids. In order for these acetyl units to be utilized for fatty acid synthesis they must be transported into the cytoplasm. The shift from fatty acid oxidation and glycolytic oxidation occurs when the need for energy diminishes. This results in reduced oxidation of acetyl-CoA in the TCA cycle. Under these conditions the mitochondrial acetyl units can be stored as fat for future energy demands.
Acetyl-CoA enters the cytoplasm in the form of citrate via the tricarboxylate transport system (Figure 19-3). In the cytoplasm, citrate is converted to oxaloacetate and acetyl-CoA by the ATP-driven ATP-citrate lyase (ACL) reaction. This reaction is essentially the reverse of that catalyzed by the TCA enzyme citrate synthase except it requires the energy of ATP hydrolysis to drive it forward. The resultant oxaloacetate is converted to malate by malate dehydrogenase (MDH).
FIGURE 19-3: Pathway for the movement of acetyl-CoA units from within the mitochondrion to the cytoplasm for use in lipid and cholesterol biosynthesis. Note that the cytoplasmic malic enzyme–catalyzed reaction generates NADPH which can be used for reductive biosynthetic reactions such as those of fatty acid and cholesterol synthesis. Reproduced with permission of themedicalbiochemistrypage, LLC.
The malate produced by this pathway can undergo oxidative decarboxylation by malic enzyme. The coenzyme for this reaction is NADP+-generating NADPH. The advantage of this series of reactions for converting mitochondrial acetyl-CoA into cytoplasmic acetyl-CoA is that the NADPH produced by the malic enzyme reaction can be a major source of reducing cofactor for the FAS activities.
ATP-Citrate Lyase: Role in Epigenetics
Epigenetics refers to the generation of a particular phenotype due to effects “on” a gene as opposed to effects “by” a gene. Acetylation of histones in chromatin is a major mechanism of epigenetic regulation of gene expression. Histone acetylation relies on acetyl-CoA synthetase enzymes that use acetate to produce acetyl-CoA. However, humans have only low extracellular concentrations of acetate such that there is a dependence on glucose metabolism for generation of acetyl-CoA for histone acetylation. ATP-citrate lyase (ACL) is the critical enzyme necessary to convert glucose-derived citrate into acetyl-CoA. Indeed, ACL is required for increases in histone acetylation in response to growth factor stimulation as well as during differentiation, and glucose availability can affect histone acetylation in an ACL-dependent manner. ACL activity is, therefore, required to link growth factor–induced increases in nutrient metabolism to the regulation of histone acetylation and ultimately gene expression patterns. Nutrient-responsive histone acetylation selectively alters the expression of genes required to reprogram intracellular metabolism to use glucose for ATP production and macromolecular synthesis.
Regulation of Fatty Acid Synthesis
There are 2 major isoforms of ACC in mammalian tissues. These are identified as ACC1 and ACC2. ACC1 is strictly cytosolic and is enriched in liver, adipose tissue, and lactating mammary tissue. ACC2 expression occurs in the heart, liver, and skeletal muscle. ACC2 has an N-terminal extension that contains a mitochondrial targeting motif and is found associated with carnitine palmitoyltransferase I (CPT I) involved in fatty acid oxidation (Chapter 25). The synthesis of malonyl-CoA by ACC2 allows for rapid regulation of CPT I.
Both isoforms of ACC are allosterically activated by citrate and inhibited by palmitoyl-CoA and other short- and long-chain fatty acyl-CoAs. Citrate triggers the polymerization of ACC1 which leads to significant increases in its activity. Although ACC2 does not undergo significant polymerization (presumably due to its mitochondrial association), it is allosterically activated by citrate. Glutamate and other dicarboxylic acids can also allosterically activate both ACC isoforms.
ACC activity is also regulated by phosphorylation. Both ACC1 and ACC2 contain at least 8 sites that undergo phosphorylation. The sites of phosphorylation in ACC2 have not been as extensively studied as those in ACC1. Phosphorylation of ACC1 by AMPK (5′ adenosine monophosphate–activated protein kinase) leads to inhibition of the enzyme. Glucagon-mediated increases in PKA activity lead to phosphorylation of both ACC isoforms where ACC2 is a better substrate for PKA than is ACC1. The activating effects of insulin on ACC are complex and not completely resolved. It is known that insulin leads to the dephosphorylation of the AMPK-phosphorylated sites in heart ACC but not in hepatocytes or adipose tissues. At least a portion of the ACC-activating effects of insulin are related to decreases in cAMP levels (Figure 19-4).
FIGURE 19-4: Regulation of acetyl-CoA carboxylase by phosphorylation/dephosphorylation. The enzyme is inactivated by phosphorylation by AMP-activated protein kinase (AMPK), which in turn is phosphorylated and activated by AMP-activated protein kinase kinase (AMPKK). Glucagon (and epinephrine) increase cAMP, and thus activate this latter enzyme via cAMP-dependent protein kinase. The kinase kinase enzyme is also believed to be activated by acyl-CoA. Insulin activates acetyl-CoA carboxylase via dephosphorylation of AMPK. Murray RK, Bender DA, Botham KM, Kennelly PJ, Rodwell VW, Weil PA. Harper’s Illustrated Biochemistry, 29th ed. New York, NY: McGraw-Hill; 2012.
Long-term regulation of fatty acid synthesis occurs at the level of gene expression primarily controlled by the circulating levels of insulin and glucagon. Insulin stimulates both ACC and FAS synthesis, whereas starvation leads to decreased synthesis of these enzymes.
High-Yield Concept
ACC is the rate-limiting and highly regulated step in fatty acid synthesis.
ChREBP: Master Lipid Regulator in the Liver
When glycogen stores are maximal in the liver, excess glucose is diverted into the lipid synthesis pathway. Glucose is catabolized to acetyl-CoA and the acetyl-CoA is used for de novo fatty acid synthesis. The fatty acids are then incorporated into triglycerides and exported from hepatocytes as VLDL (very-low-density lipoprotein) and ultimately stored as triglycerides in adipose tissue. A diet rich in carbohydrates leads to stimulation of both the glycolytic and lipogenic pathways. Genes encoding glucokinase (GK) and liver pyruvate kinase (L-PK) of glycolysis and ATP-citrate lyase (ACL), ACC, and FAS of lipogenesis are regulated by modulation of their transcription rates. These genes contain glucoseor carbohydrate-response elements (ChoREs) that are responsible for their transcriptional regulation.
One transcription factor that exerts control over glucose and lipid homeostasis is sterol-response element-binding protein (SREBP), in particular SREBP-1c (see Chapter 26). Another critical transcription factor regulating glucose and lipid homeostasis is carbohydrate-responsive element-binding protein, ChREBP.
The kinases PKA and AMPK both phosphorylate ChREBP rendering it inactive. Under conditions of low (basal) glucose concentration, ChREBP is phosphorylated and resides in the cytosol. When glucose levels rise, protein phosphatase 2A delta (PP2Aδ) removes phosphates from ChREBP allowing it to migrate to the nucleus. PP2Aδ is activated by xylulose 5-phosphate, an intermediate in the pentose phosphate pathway (PPP) (Chapter 15).
Genes whose expression is under control of ChREBP activity include L-PK, ACC, and FAS as well as glycerol 3-phosphate acyltransferase (GPAT) and Δ9-stearoly-CoA desaturase 1 (SCD1). GPAT is the enzyme that esterifies glycerol 3-phospate–generating lysophosphatidic acid which is the first step in the synthesis of triglycerides (Chapter 20) and SCD1 is the rate-limiting enzyme involved in the synthesis of the major monounsaturated fatty acids, oleic acid (18:1) and palmitoleic acid (16:1), as discussed below.
The liver X receptors (LXRs) are members of the steroid/thyroid hormone superfamily of intracellular receptors that migrate to the nucleus upon ligand binding and regulate gene expression by binding to specific target sequences (Chapter 40). The LXRs are important regulators of the lipogenic gene expression profile, in part due to LXR-mediated regulation of expression of the ChREBP gene. In addition, glucose itself can bind and activate LXRs, thereby mediating regulation of the lipogenic pathway directly (Figure 19-5).
FIGURE 19-5: Role of ChREBP in the modulation of lipid and glucose homeostasis. Increased entry of glucose into the cell results in enhanced oxidation in the PPP, resulting in increased levels of xylulose-5-phosphate (X5P). X5P activates the phosphatase PP2Aδ which removes inhibitory phosphorylations on ChREBP both in the cytosol and the nucleus. Active ChREBP then can turn on the expression of numerous genes involved in the homeostasis of glucose and lipid metabolism in the liver. Activation of LXRα, by lipid ligands, results in increased expression of ChREBP in the liver, which in turn can lead to furhter modulation of lipid and glucose homeostasis. GPAT is glycerol-3-phosphate acyltransferase. SCD1 is stearoyl-CoA desaturase. L-PK is liver pyruvate kinase. ACC is acetyl-CoA carboxylase. FAS is fatty acid synthase. MUFA is monounsaturated fatty acid. The PKA and AMPK sites of phosphorylation in ChREBP are indicated where S is serine and T is threonine and the numbers refer to the specific amino acid in the ChREBP protein. PPP=pentose phosphate pathway. Reproduced with permission of themedicalbiochemistrypage, LLC.
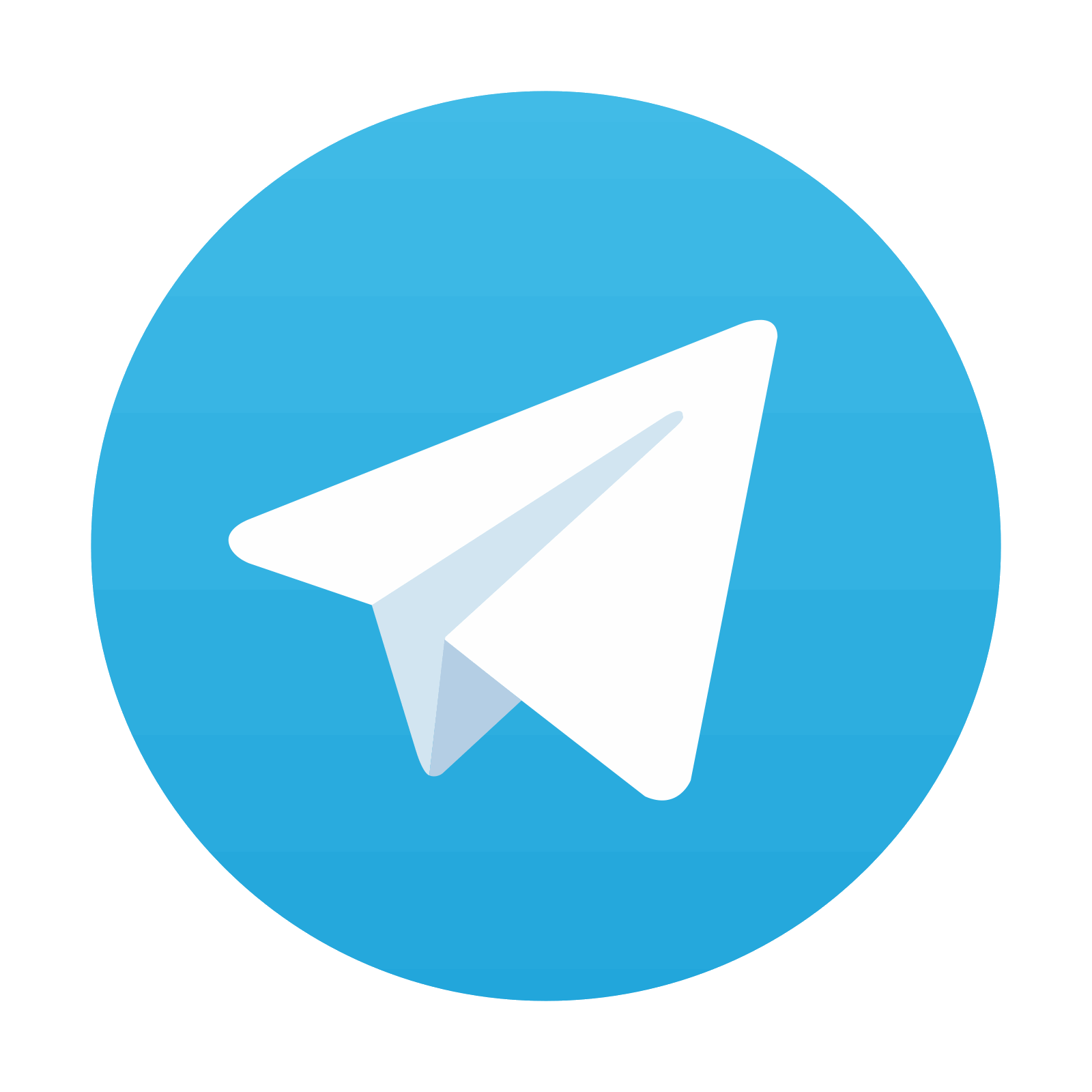
Stay updated, free articles. Join our Telegram channel
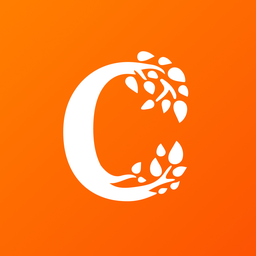
Full access? Get Clinical Tree
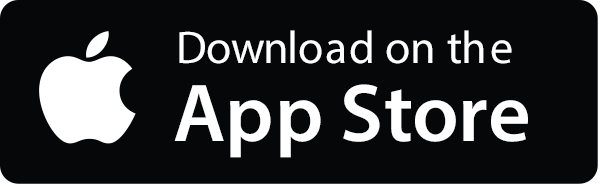
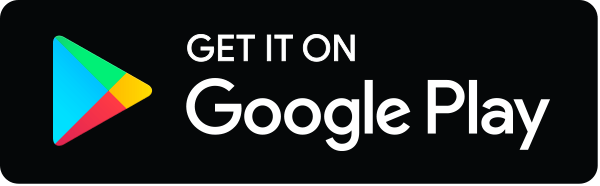