CHAPTER OUTLINE
Enzymes of Ethanol Metabolism: ADHs
Enzymes of Ethanol Metabolism: ALDHs
Acetate From EtOH Metabolism and Fatty Liver
Microsomal Ethanol Oxidation System
Ethanol Metabolism and Alcoholism
High-Yield Terms
Alcohol dehydrogenase (ADH): any of a family of enzymes that catalyze the interconversion of alcohols and aldehydes or ketones; humans express multiple forms of ADH from at least 7 distinct genes
Aldehyde dehydrogenase (ALDH): any of a family of enzymes that catalyze the oxidation of aldehydes, 2 primary enzymes, ALDH1A1 and ALDH2, involved in metabolizing acetaldehyde generated during ethanol oxidation
Microsomal ethanol-oxidizing system: inducible ethanol-metabolizing system employing a cytochrome P450 enzyme (CYP2E1)
Hepatic steatosis: condition of fatty liver that can result from excess alcohol consumption
Nonalcoholic fatty liver disease (NAFLD): one cause of a fatty liver (steatosis) not due to excessive alcohol use, most common form of chronic liver disease; obesity and activation of CYP2E1 are major contributors to development of NAFLD and NASH
Nonalcoholic steatohepatitis (NASH): progression of NAFLD to state of inflammation and fibrosis, the major feature in NASH is fat in the liver, along with inflammation and cellular damage; obesity and activation of CYP2E1 are major contributors to development of NAFLD and NASH
Kupffer cell: liver resident macrophages that contribute to inflammatory responses in response to increased reactive oxygen species generation during ethanol metabolism
It should be pointed out that humans evolved to express multiple ADH genes and isoforms, not for metabolism of ethanol, but to metabolize naturally occurring alcohols found in foods as well as those produced by intestinal bacteria. As an example, one form of ADH (encoded by the ADH7 gene) is responsible for the metabolism of not only ethanol but also retinol to retinaldehyde, which is the form of vitamin A necessary for vision.
Ethanol-Metabolizing Pathways
Ethanol is a small 2-carbon alcohol that, due to its small size and alcoholic hydroxyl group, is soluble in both aqueous and lipid environments. This allows ethanol to freely pass from body fluids into cells. Since the portal circulation from the gut passes first through the liver, the bulk of ingested alcohol is metabolized in the liver. The process of ethanol oxidation involves at least 3 distinct enzymatic pathways. The most significant pathway, responsible for the bulk of ethanol metabolism, is that initiated by alcohol dehydrogenase, ADH. ADH is an NAD+-requiring enzyme expressed at high concentrations in hepatocytes. Animal cells (primarily hepatocytes) contain cytosolic ADH, which oxidizes ethanol to acetaldehyde. Acetaldehyde then enters the mitochondria where it is oxidized to acetate by one of several aldehyde dehydrogenases (ALDHs). A cytosolic ALDH exists but is responsible for only a minor amount of acetaldehyde oxidation.
The second major pathway for ethanol metabolism is the microsomal ethanol-oxidizing system (MEOS), which involves the NADPH-dependent cytochrome P450 enzyme, CYP2E1. The MEOS pathway is induced in individuals who chronically consume alcohol.
The third pathway involves a nonoxidative pathway catalyzed by fatty acid ethyl ester (FAEE) synthase. This latter pathway results in the formation of fatty acid ethyl esters and takes place primarily in the liver and pancreas, both of which are highly susceptible to the toxic effects of alcohol.
Oxidation of ethanol can also occur in peroxisomes via the activity of catalase. However, this oxidation pathway requires the presence of a hydrogen peroxide (H2O2)-generating system and as such plays no major role in alcohol metabolism under normal physiological conditions (Figure 18-1).
FIGURE 18-1: Reactions of ethanol metabolism. Reproduced with permission of themedicalbiochemistrypage, LLC.
Enzymes of Ethanol Metabolism: ADHs
In humans there are multiple isoforms of ADH encoded for by 7 different ADH genes. All human ADH genes are members of a large family of enzymes known as the medium-chain dehydrogenase/reductase (MDR) superfamily. Functional ADH exists as either a homo- or a heterodimer and the active enzymes are divided into 5 distinct classes denoted I to V.
The hepatic forms of ADH are derived from the protein subunits encoded by the class I genes: ADH1A, ADH1B, and ADH1C. The α-, β-, and γ-subunits encoded by these 3 genes, respectively, can form homo- and heterodimers as indicated earlier. These ADH isoforms account for the vast majority of ethanol oxidation in the liver. As a consequence of single-nucleotide polymorphisms (SNPs) in several of the ADH genes, there are isoforms derived from the same gene that exhibit different kinetic characteristics. For example, as shown in Table 18-1 there are 3 known polymorphisms in the ADH1B gene and 2 in the ADH1C gene. The consequences of these alleles are ADH enzymes with much higher turnover rates.
Enzymes of Ethanol Metabolism: ALDHs
There are 2 primary ALDH genes in humans that are responsible for the oxidation of acetaldehyde generated during the oxidation of ethanol. These genes are identified as ALDH1A1 and ALDH2 and encode the ALDH1 and ALDH2 enzymes, respectively. The ALDH1 protein is a cytosolic enzyme, while the ALDH2 protein resides in the mitochondria. The bulk of acetaldehyde oxidation occurs in the mitochondria via ALDH2. However, some oxidation will occur in the cytosol via ALDH1 as a means to help control overall levels of acetaldehyde. This latter fact is most apparent in individuals with ALDH2 alleles that exhibit low to no acetaldehyde-oxidizing capacity. Several ALDH2 polymorphisms are known to exist in various populations. Indeed, the most highly studied gene variations in alcohol-metabolizing enzymes are those in the ALDH2 gene. The ALDH2*2 allele encodes a nearly inactive ALDH2 enzyme. This particular ALDH2 allele is responsible for the ease with which many individuals of Asian descent become intoxicated by alcohol consumption and this fact is due to the reduced rate of ethanol metabolism. In addition, because the levels of acetaldehyde in the blood of these individuals rises rapidly following alcohol consumption, it leads to the highly adverse reactions to this compound that includes severe flushing, nausea, and tachycardia.
Due to the negative physiological effects of the ALDH2*2 allele, even heterozygous individuals are strongly protected against alcohol dependence.
Acetate From EtOH Metabolism and Fatty Liver
Under normal conditions, the levels of acetate in human serum is less than 0.2 mM. Therefore, the roles of acetate metabolism in mammals under normal physiological conditions remain to be established. Normal physiological sources of acetate include bacterial fermentation in the colon, which increases significantly when consuming a high-fiber diet. This intestinal acetate enters the portal circulation and is taken up by the liver where it is converted to acetyl-CoA. Intracellular generation of acetate is the consequence of the ubiquitously expressed cytosolic enzyme acetyl-CoA hydrolase. The acetate can then be salvaged by reactivation to acetyl-CoA. In the nervous system, the neurotransmitter acetylcholine (ACh) is degraded to acetate by acetylcholinesterase. In order to replenish the pool of Ach, the acetate must be reactivated to acetyl-CoA so that it can participate in the choline acetyltransferase-catalyzed reaction. Acetate is also generated within the nucleus of all cells via the action of histone deacetylases (HDACs). As with the other sources of acetate, this nuclear acetate must be reactivated before it can be oxidized or reused. Under conditions of prolonged starvation and in Type 1 diabetes, the endogenous pathways of acetate production are the main sources for serum acetate. Following the consumption of ethanol, acetate levels can be elevated by as much as 20-fold.
High-Yield Concept
Humans express cytosolic and mitochondrial acetyl-CoA synthetases, AceCS1 and AceCS2, respectively. Expression of AceCS1 is under the control of SREBP-1c, which is itself transcriptionally regulated by PGC-1α.
Acetate, from whatever source, is converted to acetyl-CoA by ATP-dependent acetyl-CoA synthetases (AceCS) by the following reaction:
The primary causes of fatty liver syndrome (hepatic steatosis), induced by excess alcohol consumption are the altered NADH/NAD+ levels that in turn inhibit gluconeogenesis, inhibit fatty acid oxidation, and inhibit the activity of the TCA cycle. Each of these inhibited pathways results in the diversion of acetyl-CoA into de novo fatty acid synthesis. Ethanol has also been shown to activate SREBP-1c, which results in the transcriptional activation of numerous genes involved in lipogenesis (see Chapter 19). However, given that large amounts of acetate are generated via ethanol metabolism in the liver, this can be a significant contributor to the overall pool of acetyl-CoA utilized as the precursor for fatty acid and cholesterol biosynthesis.
Microsomal Ethanol Oxidation System
Under conditions of chronic alcohol ingestion, the increased level of ethanol metabolism cannot be accounted for solely via the ADH- and ALDH-catalyzed reactions. Studies in animals demonstrated that the alcohol-induced increase in metabolism was associated with hepatic smooth endoplasmic reticulum (SER; also referred to as microsomal membranes). This ethanol-induced metabolic system is, therefore, referred to as the microsomal ethanol-oxidation system (MEOS). The MEOS contains a cytochrome-P450 activity that is distinct from ADH and is designated as CYP2E1. Induction of CYP2E1 mRNA and enzyme activity ranges from 4- to 10-fold in the liver following alcohol intake (Figure 18-2).
FIGURE 18-2: Physiological and toxic activities associated with hepatic CYP2E1. Reproduced with permission of themedicalbiochemistrypage, LLC.
Given that the MEOS pathway for ethanol metabolism is induced in chronic alcoholics, the enhanced ethanol metabolism likely contributes to alcoholics’ metabolic tolerance for ethanol, which in turn promotes further alcohol consumption. The activity of CYP2E1 is also essential in the metabolism of several xenobiotics. Therefore, the increased level of expression of this enzyme in alcoholics can have a significant impact on the production of toxic metabolites and, thus, contributes to ethanol-induced liver injury. Metabolism of ethanol by CYP2E1 also results in a significant increase in free radical and acetaldehyde production, which, in turn, diminish reduced glutathione (GSH) and other defense systems against oxidative stress leading to further hepatocyte damage.
Increased activity of CYP2E1 results in accelerated production of lipid hydroperoxides (LOOH in the Figure 18-2) and is a significant contributor to the development of nonalcoholic fatty liver disease, NAFLD and nonalcoholic steatohepatitis, NASH. Both NAFLD and NASH are commonly associated with obesity, Type 2 diabetes, and hyperlipidemia.
Ethanol Metabolism and Alcoholism
As indicated in Table 18-1, several alleles exist for the ADH1B and ADH1C genes. Several of these alleles have been correlated with either an increased or decreased propensity toward alcohol abuse or dependence. Of clinical significance is the fact that these associations between ADH and ALDH alleles and alcoholism are the strongest and most widely reproduced associations of any gene with this disorder.
The class I ADH and ALDH2 genes play a central role in alcohol metabolism. Polymorphisms in the genes encoding ADH and ALDH produce enzymes that vary in activity. These genetic variations have been associated with an individual’s susceptibility to developing alcoholism and alcohol-related tissue damage. The ADH1B alleles occur at different frequencies in different populations. For example, the ADH1B*1 form is found predominantly in Caucasian and Black populations, whereas ADH1B*2 frequency is higher in Chinese and Japanese populations and in 25% of people with Jewish ancestry. Also, African Americans and Native Americans with the ADH1B*3 allele metabolize alcohol at a faster rate than those with ADH1B*1.
Although several ALDH isozymes have been identified, only the cytosolic ALDH1 and the mitochondrial ALDH2 metabolize acetaldehyde. There is one significant genetic polymorphism of the ALDH2 gene, resulting in allelic variants ALDH2*1 and ALDH2*2, which are virtually inactive. ALDH2*2 is present in about 50% of the Taiwanese, Chinese, and Japanese populations and shows virtually no acetaldehyde-metabolizing activity in vitro. ALDH2*2 heterozygotes, and most significantly homozygotes, show increased acetaldehyde levels after alcohol consumption and, therefore, experience significant negative physiological responses to alcohol intake.
Because polymorphisms of ADH and ALDH2 play an important role in determining peak blood acetaldehyde levels and voluntary ethanol consumption, they also influence vulnerability to alcohol dependence. A fast ADH or a slow ALDH are expected to elevate acetaldehyde levels and, thus, reduce the tolerance to alcohol consumption.
Acute and Chronic Effects of Ethanol Metabolism
The primary acute effects of ethanol consumption are the result of the altered NADH:NAD+ ratio that is the consequence of both the ADH- and ALDH-catalyzed reactions. Acute effects resulting from ethanol metabolism are also due to the fact that acetaldehyde forms adducts with proteins, nucleic acids, and other compounds resulting in impaired activity of the affected compounds. Additional acute consequences of ethanol metabolism include oxygen deficits (ie, hypoxia) in the liver and the formation of highly reactive oxygen-containing molecules (ie, reactive oxygen species, ROS) that can damage other cell components.
The NADH produced in the cytosol by ADH must be reduced back to NAD+ via either the malate-aspartate shuttle or the glycerol-phosphate shuttle. Thus, the ability of an individual to metabolize ethanol is dependent upon the capacity of hepatocytes to carry out either of these 2 shuttles, which in turn is affected by the rate of the TCA cycle in the mitochondria. The rate of flux through the TCA cycle is itself being negatively affected by the NADH produced by the ADH and ALDH reactions.
The reduction in NAD+ impairs the flux of glucose through glycolysis at the glyceraldehyde-3-phosphate dehydrogenase reaction, thereby limiting energy production. Additionally, there is an increased rate of hepatic lactate production due to the effect of increased NADH on the direction of the hepatic lactate dehydrogenase (LDH) reaction. This reversal of the LDH reaction in hepatocytes diverts pyruvate (and also alanine) from gluconeogenesis, leading to a reduction in the capacity of the liver to deliver glucose to the blood resulting in hypoglycemia.
In addition to the negative effects of the altered NADH:NAD+ ratio on hepatic gluconeogenesis, fatty acid oxidation is also reduced as this process requires NAD+ as a cofactor. Concomitant with reduced fatty acid oxidation is enhanced fatty acid synthesis and increased triglyceride production by the liver. In the mitochondria, the production of acetate from acetaldehyde leads to increased levels of acetyl-CoA. Since the increased generation of NADH also reduces the activity of the TCA cycle, the acetyl-CoA is diverted to fatty acid synthesis. The reduction in cytosolic NAD+ leads to reduced activity of glycerol-3-phosphate dehydrogenase (in the glycerol 3-phosphate to DHAP direction), resulting in increased levels of glycerol 3-phosphate which is the backbone for the synthesis of the triglycerides. Both of these 2 events lead to fatty acid deposition in the liver leading to fatty liver syndrome and excessive levels of lipids in the blood, referred to as hyperlipidemia (Figure 18-3).
FIGURE 18-3: Acute effects of ethanol metabolism on liver functions. Reproduced with permission of themedicalbiochemistrypage, LLC.
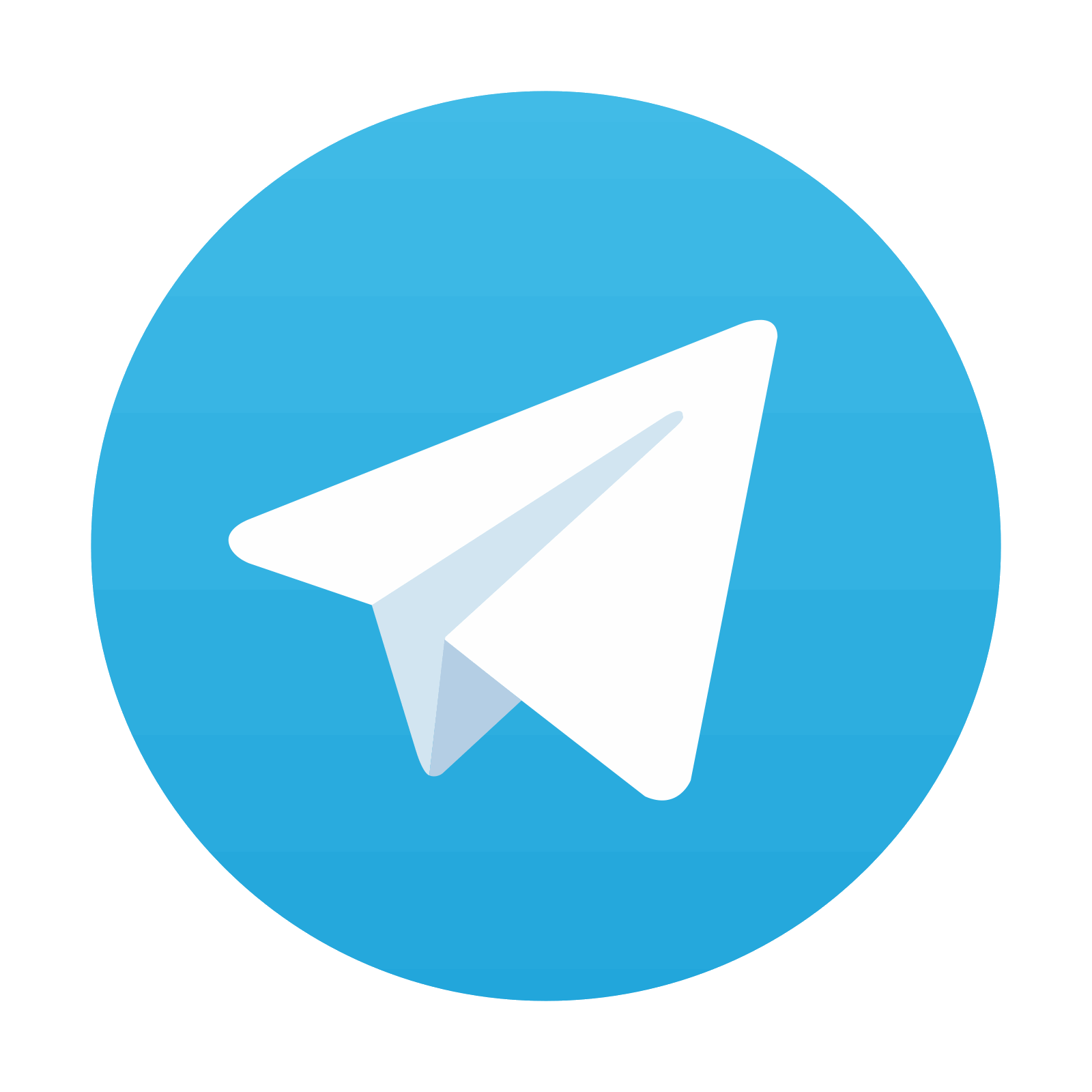
Stay updated, free articles. Join our Telegram channel
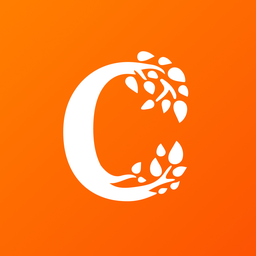
Full access? Get Clinical Tree
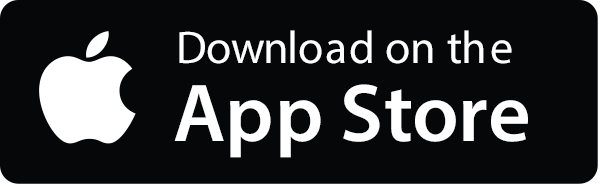
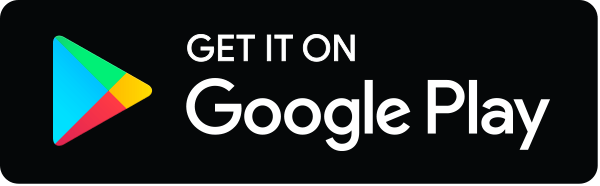