High-Yield Terms
Glycosidic linkage: covalent bond that joins a carbohydrate (sugar) molecule to another group such as another sugar typical of the bonds between glucose molecules in glycogen
Glycogenin: protein with self-glycosylating activity that serves as the primer molecule for initiation of glycogen synthesis
Glycogen synthase-phosphorylase kinase: this enzyme is critical for the regulation of the flux of glucose into and out of glycogen as it reciprocally regulates the 2 key enzymes of glycogenolysis and glycogen synthesis
Glycogen storage disease: disorders that result from defects in genes encoding enzymes involved in the process of glycogen synthesis or breakdown, primarily affecting muscles and liver
Glycogen Composition
Stores of readily available glucose, used to supply the tissues with an oxidizable energy source, are found principally in the liver, as glycogen. Glycogen is a polymer of glucose residues linked by α-(1,4)- and α-(1,6)-glycosidic bonds. A second major source of stored glucose is the glycogen of skeletal muscle. However, muscle glycogen is not generally available to other tissues, because muscle lacks the enzyme glucose 6-phosphatase (Figure 14-1).
FIGURE 14-1: Section of glycogen showing α-1,4- and α-1,6-glycosidic linkages. Reproduced with permission of themedicalbiochemistrypage, LLC.
The major site of daily glucose consumption (75%) is within the brain. The remainder of it is utilized mostly by erythrocytes, skeletal muscle, and heart muscle. The body obtains glucose either directly from the diet or from amino acids and lactate via gluconeogenesis (see Chapter 13). Glucose obtained from these 2 primary sources either remains soluble in the body fluids or is stored as the glucose polymer, glycogen. Glycogen is considered the principal storage form of glucose and is found mainly in liver and muscle, with kidney and intestines adding minor storage sites. With up to 10% of its weight as glycogen, the liver has the highest specific content of any body tissue. Muscle has a much lower amount of glycogen per unit mass of tissue, but since the total mass of muscle is so much greater than that of liver, total glycogen stored in muscle is about twice that of liver. Stores of glycogen in the liver are considered the main buffer of blood glucose levels (Table 14-1).
Glycogen Synthesis (Glycogenesis)
De novo glycogen synthesis is initiated by the attachment of the first glucose residue to a protein known as glycogenin. Glycogenin has the unusual property of catalyzing its own glycosylation, attaching C–1 of a uridine diphosphate (UDP) glucose to a tyrosine residue on the enzyme. The attached glucose then serves as the primer required by glycogen synthase to attach additional glucose molecules.
Synthesis of glycogen from glucose is carried out by the enzyme glycogen synthase. This enzyme utilizes UDP glucose as one substrate and the nonreducing end of glycogen as another. The activation of glucose is carried out by the enzyme UDP glucose pyrophosphorylase. This enzyme exchanges the phosphate on C–1 of glucose 1-phosphate for UDP. The UDP is subsequently released during the glycogen synthase-mediated incorporation of glucose into glycogen. The α-1,6 branches in glucose are introduced by amylo-(1,4–1,6)-transglycosylase, more commonly just called branching enzyme. This enzyme transfers a terminal fragment of 6 to 7 glucose residues (from a polymer at least 11 glucose residues long) to an internal glucose residue at the C–6 hydroxyl position (Figures 14-2 and 14-3).
FIGURE 14-2: Enzymes necessary to activate glucose for incorporation into glycogen via glycogen synthase. Reproduced with permission of themedicalbiochemistrypage, LLC.
FIGURE 14-3: The biosynthesis of glycogen. The mechanism of branching as revealed by feeding 14C-labeled glucose and examining liver glycogen at intervals.) Murray RK, Bender DA, Botham KM, Kennelly PJ, Rodwell VW, Weil PA. Harper’s Illustrated Biochemistry, 29th ed. New York, NY: McGraw-Hill; 2012.
Regulation of Glycogen Synthesis
Glycogen synthase is a tetrameric enzyme consisting of 4 identical subunits. The liver and muscle glycogen synthase proteins are derived from different genes and share only 46% amino acid identity. The activity of glycogen synthase is influenced by phosphorylation of serine residues in the subunit proteins as well as via allosteric regulation. Phosphorylation of glycogen synthase reduces its activity toward UDP glucose. When phosphorylated, glycogen synthase activity can be increased by binding the allosteric activator, glucose 6-phosphate (G6P). Activation by G6P does not occur when glycogen synthase is not phosphorylated. The 2 forms of glycogen synthase are identified by the use of “a” and “b” such that the nonphosphorylated, and most active form, is called synthase-a and the phosphorylated less active glucose-6-phosphate–dependent form is called synthase-b.
Numerous kinases have been shown to phosphorylate and regulate both hepatic and muscle forms of glycogen synthase (Figure 14-4). At least 5 sites of phosphorylation have been identified in hepatic glycogen synthase that are the targets of at least 7 different kinases. The 7 kinases that regulate glycogen synthase activity are glycogen synthase-phosphorylase kinase (commonly just called phosphorylase kinase, PhK), PKA, PKC, glycogen synthase kinase-3 (GSK-3), calmodulin-dependent protein kinase-II (CaMPK-II), casein kinase-I (CK-I), and casein kinase-II (CK-II). Phosphorylase kinase is a multi-subunit enzyme composed of α-, β-, γ-, and δ-subunits. The α- and β-subunits are the regulatory subunits that are subject to phosphorylation. The γ-subunit is the catalytic subunit and the δ-subunit is calmodulin.
FIGURE 14-4: Control of glycogen synthase in muscle. (GSK, glycogen synthase kinase; G6P, glucose 6-phosphate; n, number of glucose residues.) Murray RK, Bender DA, Botham KM, Kennelly PJ, Rodwell VW, Weil PA. Harper’s Illustrated Biochemistry, 29th ed. New York, NY: McGraw-Hill; 2012.
When glucagon binds its receptor on hepatocytes, the receptor activates the enzyme adenylate cyclase. Activation of adenylate cyclase leads to a large increase in the formation of cAMP, which then binds to cAMP-dependent protein kinase, PKA. Binding of cAMP to the regulatory subunits of PKA leads to the release and subsequent activation of the catalytic subunits. The catalytic subunits then phosphorylate a number of target proteins such as glycogen synthase. PKA has been shown to phosphorylate glycogen synthase on at least 4 different sites. In addition, PKA activity results in an increase in the activity of phosphorylase kinase, which in turn phosphorylates glycogen synthase at one of the same sites as PKA. In addition, glucagon causes an increase in the activity of casein kinase-II (CK-II). Thus, the net effect of glucagon action on hepatocytes is activation of 3 distinct kinases that phosphorylate and thereby, inhibit glycogen synthase.
Since insulin and glucagon are counter-regulatory hormones, it should be clear that they will exert opposing effects on the rate and level of glycogen synthase phosphorylation. The action of insulin, at the level of PKA, is to increase the activity of phosphodiesterase, which hydrolyzes cAMP to AMP thereby reducing the level of active PKA. Insulin also exerts a negative effect on the activity of GSK-3 such that there is a reduced level of phosphorylation of glycogen synthase by this kinase.
Hormones and neurotransmitters that result in release of stored intracellular Ca2+ also affect negative regulation of glycogen synthase activity. The released Ca2+ ions bind to the calmodulin subunit of PhK and result in its activation leading to increased phosphorylation of glycogen synthase. Activation of α1-adrenergic receptors in skeletal muscle results in activation of PLC-β, which hydrolyzes phosphatidylinositol 4,5-bisphosphate (PIP2) releasing inositol trisphosphate (IP3) and diacylglycerol (DAG). The action of IP3 results in increased release of stored Ca2+ with the same net effect at the level of glycogen synthase. The released Ca2+ ions, in conjunction with DAG, in turn activate PKC, which phosphorylates glycogen synthase in the same domain of the enzyme that is one target for PKA and the site for CaMPK-II and CK-I phosphorylation. The overall effect of these various phosphorylation events, at the level of glycogen synthase activity, is decreased affinity for substrate (UDP glucose) and for the allosteric activator G6P.
Reactivation of glycogen synthase requires dephosphorylation, which is carried out predominately by the serine/threonine phosphatase identified as protein phosphatase-1 (PP-1). The activity of PP-1 must also be regulated so that the phosphate residues are not immediately removed. This is accomplished by the binding of PP-1 to phosphoprotein phosphatase inhibitor (PPI-1). This protein also is phosphorylated by PKA and dephosphorylated by PP-1. The phosphorylation of PPI allows it to bind to PP-1, an activity it is incapable of carrying out when not phosphorylated. When PPI binds to PP-1, the rate of PP-1-mediated phosphate removal from PPI is significantly reduced, effectively trapping PP-1 from other substrates. As is to be expected, insulin exerts an opposing effect to that of glucagon and epinephrine at the level of PP-1 activity.
The conversion of glucose 6-phosphate to free glucose occurs via the action of glucose 6-phosphatase. This enzyme is expressed in the liver, intestine, and kidney (key gluconeogenic tissues) but not in skeletal muscle. Therefore, any glucose released from glycogen stores of muscle will be oxidized in the glycolytic pathway.
Glycogenolysis
Degradation of stored glycogen, termed glycogenolysis, occurs through the action of glycogen phosphorylase (Figure 14-5). The action of phosphorylase is to phosphorolytically remove single glucose residues from α-(1,4)-linkages within the glycogen molecule. The product of this reaction is glucose 1-phosphate. The advantage of the reaction proceeding through a phosphorolytic step is that the glucose is removed from glycogen is an activated state (ie, phosphorylated) without ATP hydrolysis. The glucose 1-phosphate produced by the action of phosphorylase is converted to glucose 6-phosphate by phosphoglucomutase (PGM).
FIGURE 14-5: Phosphorylase-catalyzed reaction. Reproduced with permission of themedicalbiochemistrypage, LLC.
Glycogen phosphorylase cannot remove glucose residues from the branch points (α-1,6 linkages) in glycogen. The activity of phosphorylase ceases 4 glucose residues from the branch point. The removal of these branch-point glucose residues requires the action of debranching enzyme (also called glucan transferase), which contains 2 activities: glucotransferase and glucosidase. The transferase activity removes the terminal 3 glucose residues of one branch and attaches them to a free C–4 end of a second branch. The glucose in α-(1,6)-linkage at the branch is then removed by the action of glucosidase as free glucose (Figure 14-6).
FIGURE 14-6: Steps in glycogenolysis. Murray RK, Bender DA, Botham KM, Kennelly PJ, Rodwell VW, Weil PA. Harper’s Illustrated Biochemistry, 29th ed. New York, NY: McGraw-Hill; 2012.
High-Yield Concept
The activity of hexokinase in muscle is so high that any free glucose is immediately phosphorylated and enters the glycolytic pathway. Indeed, the precise reason for the temporary appearance of the free glucose from glycogen is the need of the skeletal muscle cell to generate energy from glucose oxidation, thereby, precluding any chance of the glucose entering the blood.
Regulation of Glycogenolysis
Glycogen phosphorylase (most commonly just phosphorylase) is a homodimeric enzyme that exists in 2 distinct conformational states: a T (for tense, less active) and R (for relaxed, more active) state. Phosphorylase is capable of binding to glycogen when the enzyme is in the R state. This conformation is enhanced by binding the allosteric activator AMP and inhibited by binding the allosteric inhibitors ATP or glucose 6-phosphate. The activity of phosphorylase is also modulated by phosphorylation. The relative activity of the unmodified phosphorylase enzyme (phosphorylase-b) is sufficient to generate enough glucose 1-phosphate for entry into glycolysis for the production of adequate amounts of ATP to maintain the normal resting activity of the cell. This is true in both liver and muscle cells.
When blood glucose levels fall, the pancreas secretes glucagon, which binds to cell surface receptors. Liver cells are the primary target for the action of this hormone. The binding of glucagon to its cell surface receptor results in activation of PKA. Of significance to this discussion is the PKA-mediated phosphorylation of phosphorylase kinase (Figure 14-7). Phosphorylation of phosphorylase kinase activates the enzyme, which in turn phosphorylates the b form of phosphorylase. Phosphorylation of phosphorylase-b greatly enhances its activity toward glycogen breakdown. This modified enzyme is called phosphorylase-a. The net result is an extremely large induction of glycogen breakdown in response to glucagon binding to cell surface receptors.
FIGURE 14-7: Control of phosphorylase in muscle. The sequence of reactions arranged as a cascade allows amplification of the hormonal signal at each step. (G6P, glucose 6-phosphate; n, number of glucose residues.) Murray RK, Bender DA, Botham KM, Kennelly PJ, Rodwell VW, Weil PA. Harper’s Illustrated Biochemistry, 29th ed. New York, NY: McGraw-Hill; 2012.
This identical cascade of events occurs in skeletal muscle cells as well, even though these cells lack the glucagon receptor. In muscle, the induction of the cascade is the result of epinephrine binding to its receptors on these cells. Epinephrine is released from the adrenal glands in response to neural signals indicating an immediate need for enhanced glucose utilization in muscle, the so-called fight or flight response.
High-Yield Concept
Within skeletal muscle cells, calcium ions play a crucial role in the regulation of glycogenolysis. Calcium ions bind to the calmodulin subunit of phosphorylase kinase. Binding induces a conformational change in calmodulin, which in turn enhances the catalytic activity of the phosphorylase kinase toward its substrate, phosphorylase-b. This activity is crucial to the enhancement of glycogenolysis in muscle cells where muscle contraction is induced via acetylcholine stimulation at the neuromuscular junction.
The effect of acetylcholine release from nerve terminals at a neuromuscular junction is to depolarize the muscle cell leading to increased release of Ca2+ stored in the sarcoplasmic reticulum, thereby activating phosphorylase kinase.
Calcium ions are also involved in the ultimate response to activation of α-adrenergic receptors on skeletal muscle. α1-adrenergic receptors are coupled to the activation of PLC-β. Activation of PLC-β leads to increased hydrolysis of membrane PIP2 and release of IP3 and DAG. IP3 binds to receptors on the surface of the endoplasmic reticulum, leading to release of Ca2+ ions. The Ca2+ ions then interact with the calmodulin subunits of phosphorylase kinase resulting in its activation. Additionally, the Ca2+ ions activate PKC in conjunction with DAG.
As described earlier for glycogen synthase, once the signals that initiated the phosphorylation of phosphorylase have abated, the phosphates must be removed to return the enzyme to basal activity. The removal of the phosphates is catalyzed primarily by PP-1 as for glycogen synthase.
Glycogen Storage Diseases
Since glycogen molecules can become enormously large, an inability to degrade glycogen can cause cells to become pathologically engorged; it can also lead to the functional loss of glycogen as a source of cell energy and as a blood glucose buffer. Although glycogen storage diseases (GSDs) are quite rare, their effects can be most dramatic. The debilitating effect of many GSDs depends on the severity of the mutation causing the deficiency. In addition, although the GSDs are attributed to specific enzyme deficiencies, other events can cause the same characteristic symptoms. The GSDs are divided into 2 primary categories: those that result principally from defects in liver glycogen homeostasis and those that represent defects in muscle glycogen homeostasis. The liver GSDs result in hepatomegaly and hypoglycemia or cirrhosis, whereas the muscle GSDs result in skeletal and cardiac myopathies and/or energy impairment (Table 14-2).
CLINICAL BOX 14-1: von GIERKE DISEASE
Type I GSD was first described in 1929 by E. von Gierke as a “hepatonephromegalia glycogenica.” For this reason, the disease is still more commonly referred to von Gierke disease. In 1952, G. Cori and C. Cori identified that the absence of glucose-6-phosphatase activity was the cause of von Gierke disease. This discovery was the first-ever identification of an enzyme defect in a metabolic disorder. Subsequent to the identification of the pathway of glucose release from glucose 6-phosphate, additional patients with similar clinical manifestations to von Gierke disease were identified. However, these patients were not deficient in glucose 6-phosphatase. These latter patients were identified as having type Ib GSD. Type Ia GSD is caused by a defect in the ER localized glucose 6-phosphatase. Type Ib disease results from defects in the glucose-6-phosphate transporter 1. Type Ic GSD was identified in 1983 and found to be the result of defects in the microsomal pyrophosphate transporter. This form of type I GSD has only been found in few cases. As shown in Figure 14-8, there are 4 enzyme activities that function in the release of free glucose in the cell, and as such there is the theoretical possibility that type Id GSD would be caused by defects in the microsomal glucose transporter. However, no individuals have been reported to exist with a defect in this latter enzyme activity. Type I GSD (von Gierke disease) is attributed to lack of glucose 6-phosphatase, G-6Pase. However, G-6Pase is localized to the luminal side of the endoplasmic reticulum (ER) membrane. In order to gain access to the phosphatase, glucose 6-phosphate must pass through a specific translocase in the ER membrane (Figure 14-8). Mutation of either the phosphatase or the translocase makes transfer of liver glycogen to the blood a very limited process. Thus, mutation of either gene leads to symptoms associated with von Gierke disease, which occurs at a rate of about 1 in 200,000 people (Figure 14-8).
FIGURE 14-8: Multistep process for the removal of phosphate from glucose 6-phosphate so that free glucose can be relased from the cell. Reproduced with permission of themedicalbiochemistrypage, LLC.
The metabolic consequences of the hepatic glucose-6-phosphate deficiency of von Gierke disease extend well beyond just the obvious hypoglycemia that result from the deficiency in liver being able to deliver free glucose to the blood. The inability to release the phosphate from glucose 6-phopsphate results in diversion into glycolysis and production of pyruvate as well as increased diversion onto the pentose phosphate pathway. The production of excess pyruvate, at levels above the capacity of the TCA cycle to completely oxidize it, results in its reduction to lactate resulting in lactic acidemia. In addition, some of the pyruvate is transaminated to alanine, leading to hyperalaninemia. Some of the pyruvate will be oxidized to acetyl-CoA, which cannot be fully oxidized in the TCA cycle and so the acetyl-CoA will end up in the cytosol where it will serve as a substrate for triglyceride and cholesterol synthesis resulting in hyperlipidemia. The oxidation of glucose 6-phosphate via the pentose phosphate pathway leads to increased production of ribose 5-phosphate, which then activates the de novo synthesis of the purine nucleotides. In excess of the need, these purine nucleotides will ultimately be catabolized to uric acid resulting in hyperuricemia and consequent symptoms of gout. The interrelationships of these metabolic pathways are shown in Figure 14-9.
FIGURE 14-9: Interrelationships of metabolic pathway disruption in von Gierke disease. Reproduced with permission of themedicalbiochemistrypage, LLC.
Patients with type I GSD can present during the neonatal period with lactic acidosis and hypoglycemia. More commonly though, infants of 3 to 4 months of age will manifest with hepatomegaly and hypoglycemic seizures. The hallmark features of this disease are hypoglycemia, lactic acidosis, hyperuricemia, and hyperlipidemia. The severity of the hypoglycemia and lactic acidosis can be such that in the past affected individuals died in infancy. Infants often have a doll-like facial appearance due to excess adipose tissue in the cheeks. In addition, patients have thin extremities, a short stature, and protuberant abdomens (due to the severe hepatomegaly).
Long-term complications are usually seen now only in adults whose disease was poorly treated early on. The main problem is associated with liver function but multiple organ systems are also involved, in particular the intestines and kidneys. Growth continues to be impaired and puberty is often delayed. Affected female patients will have polycystic ovaries but none of the other symptoms of polycystic ovarian syndrome (PCOS) such as hirsutism.
The common treatment for type I GSD is to maintain normal blood glucose concentration. With normoglycemia will come reduced metabolic disruption and a reduced morbidity associated with the disease. To attain normoglycemia, patients are usually treated in infancy with nocturnal nasogastric infusion of glucose. Total parenteral nutrition or the oral feeding of uncooked cornstarch can also achieve the desired results. In the past the prognosis for type I GSD patients was poor. However, with proper nutritional intervention, growth will improve and the lactic acidosis, cholesterol, and lipidosis will decrease.
CLINICAL BOX 14-2: POMPE DISEASE
Glycogen storage disease type II (GSDII) is also known as Pompe disease or acid maltase deficiency (AMD). This disease was originally referred to as Pompe disease since Joannes Cassianus Pompe (published in 1932) made the important observation of a massive accumulation of glycogen within the vacuoles of all tissues in a 7-month-old female infant who died suddenly from idiopathic hypertrophy of the heart. Through the investigations carried out by the Coris (Gerty T. Cori and Carl F. Cori), this disease was classified as GSD type II. GSDII is the most severe of all the GSDs. The excess storage of glycogen in the vacuoles is the consequence of defects in the lysosomal hydrolase, acid α-glucosidase, which removes glucose residues from glycogen in the lysosomes. The acid α- glucosidase gene (designated GAA) resides on chromosome 17q25 spanning 20 kb and composed of 20 exons. GSDII has been shown to be caused by missense, nonsense, and splice-site mutations, partial deletions and insertions. Some mutations are specific to certain ethnic groups. There are 3 common allelic forms of acid α-glucosidase that segregate in the general population. These forms are designated GAA1, GAA2, and GAA4. The normal function of acid α-glucosidase is to hydrolyze both α-1,4- and α-1,6-glucosidic linkages at acid pH. The activity of the enzyme leads to the complete hydrolysis of glycogen, which is its natural substrate. As would be expected from this activity, deficiency in acid α-glucosidase leads to the accumulation of structurally normal glycogen in numerous tissues, most notably in cardiac and skeletal muscle. The clinical presentation of GSDII encompasses a wide range of phenotypes but all include various degrees of cardiomegaly. Additional clinical manifestations associated with idiopathic cardiomegaly accompanied by storage of glycogen are indicative of Pompe disease. These symptoms included hepatomegaly, marked hypotonia, muscular weakness, and death before 1 year of age. The different phenotypes can be classified dependent upon age of onset, extent of organ involvement, and the rate of progression to death. The infantile-onset form is the most severe and was the phenotype described by J.C. Pompe. The other extreme of this disorder is a slowly progressing adult-onset proximal myopathic disease. The late-onset disease usually presents as late as the second to sixth decade of life and usually only involves the skeletal muscles. There is also a heterogeneous group of GSDII disorders that are classified generally by onset after early infancy and called the juvenile or childhood form. In addition to classical symptoms that can lead to a diagnosis of GSDII, analysis for the level and activity of acid α-glucosidase in muscle biopsies is used for confirmation. The infantile onset form of GSDII presents in the first few months of life. Symptoms include marked cardiomegaly, striking hypotonia (leading to the designation of “floppy baby syndrome”), and rapid progressive muscle weakness. Patients will usually exhibit difficulty with feeding and have respiratory problems that are frequently complicated by pulmonary infection. The prominent cardiomegaly that can be seen on chest x-ray is normally the first indication leading to a preliminary diagnosis of GSDII. There is currently no cure for GSDII. In 2006 the US FDA approved the use of alglucosidase alfa (Myozyme) as an enzyme replacement therapy (ERT) for treatment of infantile-onset Pompe disease. Supportive therapy with attention to treatment of respiratory function can impact the course of the disease in the late-onset form.
CLINICAL BOX 14-3: McARDLE DISEASE
Glycogen storage disease type V (GSDV) is also known as McArdle disease. This disease was originally described by B. McArdle in 1951, hence the association of his name with the disease. The disease was seen in a 30-year-old patient who was suffering from muscle weakness, pain, and stiffness following slight exercise. It was observed that blood lactate fell in this patient during exercise instead of the normal rise that would be seen. This indicated the patient had a defect in the ability to convert muscle glycogen to glucose and ultimately lactate. The identification that the deficiency causing these symptoms was the result of a muscle phosphorylase defect was not made until 1959. Glycogen phosphorylase (most commonly just called phosphorylase) exists in multiple tissue-specific isoforms. The muscle, brain, and liver forms are encoded by separate genes. The muscle form is the only isozyme found expressed in mature muscle. The clinical presentation of GSDV is usually seen in young adulthood and is characterized by exercise intolerance and muscle cramps following slight exercise. Attacks of myoglobinuria frequently accompany the muscle symptoms of GSDV. About half of GSDV patients will exhibit burgundy-colored urine after exercise. Diagnosis of muscle glycogenoses such as GSDV can be made by the observation of a lack of increased blood lactate upon ischemic exercise testing. In addition, there will be an associated large increase in blood ammonia levels. In order to distinguish GSDV from other muscle defects along the pathway from glycogen to glucose to lactate, an enzymatic evaluation of muscle phosphorylase must be done. In addition, molecular analysis for known mutations in the muscle phosphorylase gene can be accomplished using DNA extracted from leukocytes.
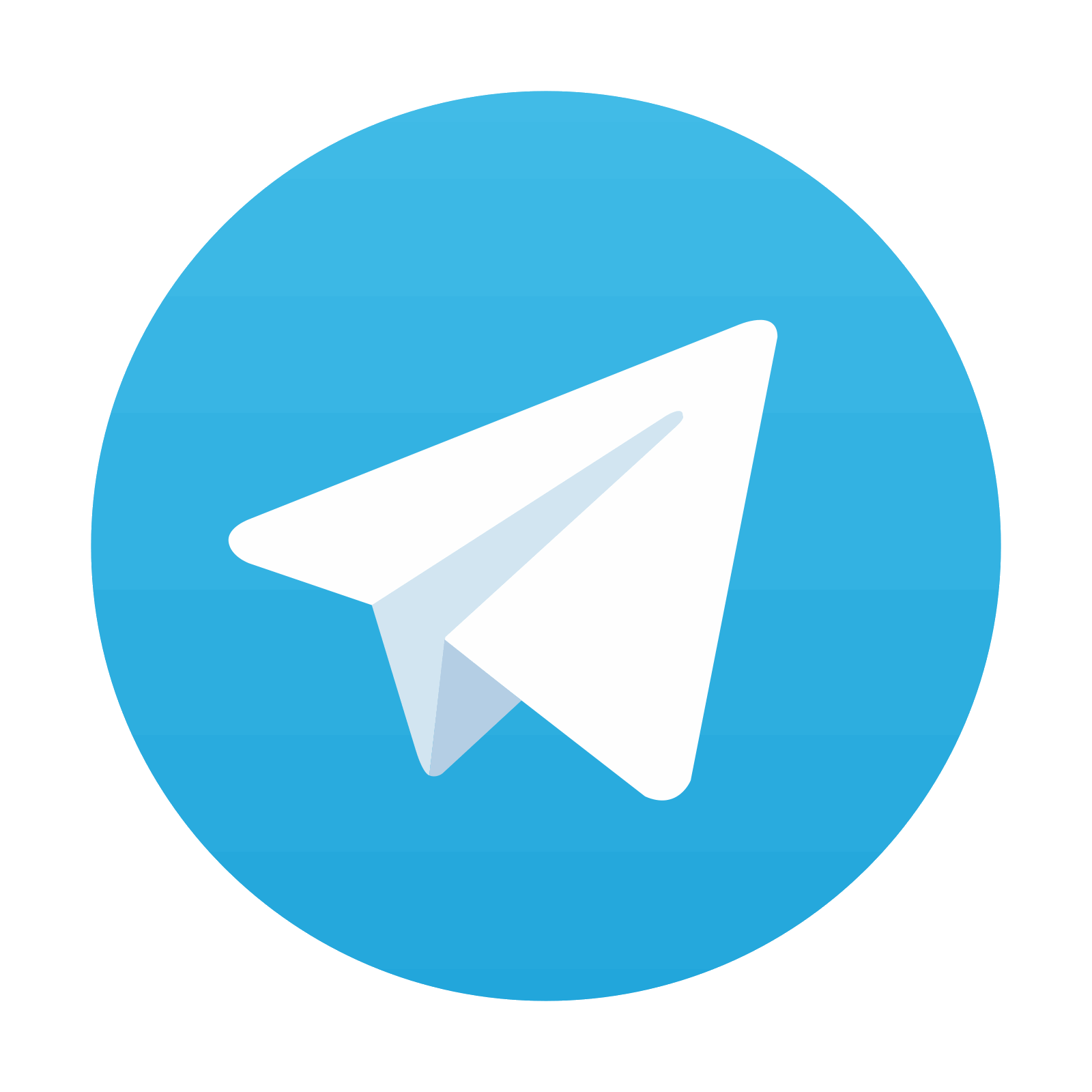
Stay updated, free articles. Join our Telegram channel
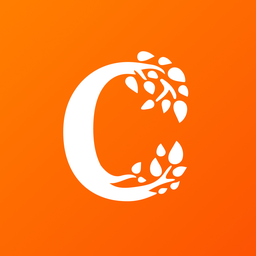
Full access? Get Clinical Tree
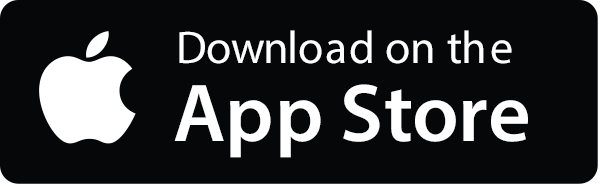
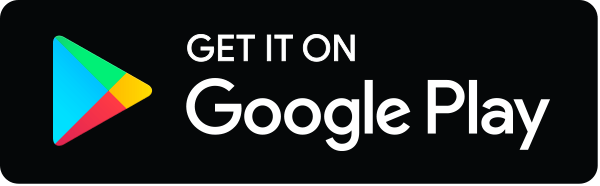