21
Introduction to the Pharmacology of CNS Drugs
Drugs acting in the central nervous system (CNS) were among the first to be discovered by primitive humans and are still the most widely used group of pharmacologic agents. These include medications used to treat a wide range of neurologic and psychiatric conditions as well as drugs that relieve pain, suppress nausea, and reduce fever, among other symptoms. In addition, many CNS-acting drugs are used without prescription to increase the sense of well-being.
Due to their complexity, the mechanisms by which various drugs act in the CNS have not always been clearly understood. In recent decades, however, dramatic advances have been made in the methodology of CNS pharmacology. It is now possible to study the action of a drug on individual neurons and even single receptors within synapses. The information obtained from such studies is the basis for several major developments in studies of the CNS. First, it is clear that nearly all drugs with CNS effects act on specific receptors that modulate synaptic transmission. While a few agents such as general anesthetics and alcohol may have nonspecific actions on membranes (although these exceptions are not fully accepted), even these non-receptor-mediated actions result in demonstrable alterations in synaptic transmission.
Second, drugs are among the most valuable tools for studying CNS function, from understanding the mechanism of convulsions to the laying down of long-term memory. Both agonists that mimic natural transmitters (and in many cases that are more selective than the endogenous substances) and antagonists are extremely useful in such studies. Third, unraveling the actions of drugs with known clinical efficacy has led to some of the most fruitful hypotheses regarding the mechanisms of disease. For example, information about the action of antipsychotic drugs on dopamine receptors has provided the basis for important hypotheses regarding the pathophysiology of schizophrenia. Studies of the effects of a variety of agonists and antagonists on γ-aminobutyric acid (GABA) receptors have resulted in new concepts pertaining to the pathophysiology of several diseases, including anxiety and epilepsy.
A full appreciation of the effects of a drug on the CNS requires an understanding of the multiple levels of brain organization, from genes to circuits to behavior. This chapter provides an introduction to the functional organization of the CNS and its synaptic transmitters as a basis for understanding the actions of the drugs described in the following chapters.
ORGANIZATION OF THE CNS
The CNS comprises the brain and spinal cord and is responsible for integrating sensory information and generating motor output and other behaviors needed to successfully interact with the environment and enhance species survival. The human brain contains about 100 billion interconnected neurons surrounded by various supporting glial cells. Throughout the CNS, neurons are either clustered into groups called nuclei or are present in layered structures such as the cerebellum or hippocampus. Connections among neurons both within and between these clusters form the circuitry that regulates information flow through the CNS.
Neurons
Neurons are electrically excitable cells that process and transmit information via an electrochemical process. There are many types of neurons in the CNS, and they are classified in the following ways: by function, by location, and by the neurotransmitter they release. The typical neuron possesses a cell body (or soma) and specialized processes called dendrites and axons (Figure 21–1). Dendrites, which form highly branched complex dendritic “trees,” receive and integrate the input from other neurons and conduct this information to the cell body. The axon carries the output signal of a neuron from the cell body, sometimes over long distances. Neurons may have hundreds of dendrites but generally have only one axon, though axons may branch distally to contact multiple targets. The axon terminal makes contact with other neurons at specialized junctions, called synapses, where neurotransmitter chemicals are released that interact with receptors on other neurons.
FIGURE 21–1 Neurons and glia in the CNS. A typical neuron has a cell body (or soma) that receives the synaptic responses from the dendritic tree. These synaptic responses are integrated at the axon initial segment, which has a high concentration of voltage-gated sodium channels. If an action potential is initiated, it propagates down the axon to the synaptic terminals, which contact other neurons. The axon of long-range projection neurons is insulated by a myelin sheath derived from specialized membrane processes of oligodendrocytes, analogous to the Schwann cells in the peripheral nervous system. Astrocytes perform supportive roles in the CNS, and their processes are closely associated with neuronal synapses. (see Figures 21-4 and 21-7).
Neuroglia
In addition to neurons, there are a large number of non-neuronal support cells, called neuroglia or glia, that perform a variety of essential functions in the CNS. Astrocytes are the most abundant glial cells in the brain and play homeostatic support roles, including providing metabolic nutrients to neurons and maintaining extracellular ion concentrations. In addition, astrocyte processes are closely associated with neuronal synapses where they are involved in the removal and recycling of neurotransmitters after release and play increasingly appreciated roles in regulating neurotransmission (see below).
Oligodendrocytes are cells that wrap around the axons of projection neurons in the CNS forming the myelin sheath (Figure 22–1). Similar to the Schwann cells in peripheral neurons, the myelin sheath created by the oligodendrocytes insulates the axons and increases the speed of signal propagation. Damage to oligodendrocytes occurs in multiple sclerosis and thus is a target of drug discovery efforts.
Microglia are specialized macrophages derived from the bone marrow that are found in the CNS and are the major immune defense system in the brain. These cells are actively involved in neuroinflammatory processes in many pathological states including neurodegenerative diseases.
Blood-Brain Barrier
The blood-brain barrier (BBB) is a protective functional separation of the circulating blood from the extracellular fluid of the CNS that limits the penetration of substances, including drugs. This separation is accomplished by the presence of tight junctions between the capillary endothelial cells as well as a surrounding layer of astrocyte end-feet. Therefore, to enter the CNS, drugs must either be highly hydrophobic or engage specific transport mechanisms. For example, the second-generation antihistamines cause less drowsiness because they were developed to be significantly more polar than older antihistamines, limiting their crossing of the BBB (see Chapter 16). Many nutrients, such as glucose and the essential amino acids, have specific transporters that allow them to cross the BBB. L-DOPA, a precursor of the neurotransmitter dopamine, can enter the brain using an amino acid transporter, whereas dopamine cannot cross the BBB. Thus, an orally administered drug, L-DOPA, but not dopamine, can be used to boost CNS dopamine levels in the treatment of Parkinson’s disease. Some parts of the brain, the so-called circumventricular organs, lack a normal BBB. These include regions that sample the blood, such as the area postrema vomiting center, and regions that secrete neurohormones into the circulation.
ION CHANNELS & NEUROTRANSMITTER RECEPTORS
The membranes of neurons contain two types of channels defined on the basis of the mechanisms controlling their gating (opening and closing): voltage-gated and ligand-gated channels (Figure 21–2A and B). Voltage-gated channels respond to changes in the membrane potential of the cell. The voltage-gated sodium channel described in Chapter 14 for the heart is an example of this type of channel. In nerve cells, these channels are highly concentrated on the initial segment of the axon (Figure 21–1), which initiates the all-or-nothing fast action potential and along the length of the axon where they propagate the action potential to the nerve terminal. There are also many types of voltage-sensitive calcium and potassium channels on the cell body, dendrites, and initial segment, which act on a much slower time scale and modulate the rate at which the neuron discharges. For example, some types of potassium channels opened by depolarization of the cell result in slowing of further depolarization and act as a brake to limit further action potential discharge. Plant and animal toxins that target various voltage-gated ion channels have been invaluable for studying the functions of these channels (see Box: Natural Toxins: Tools for Characterizing Ion Channels; Table 21–1).
TABLE 21–1 Some toxins used to characterize ion channels.
FIGURE 21–2 Types of ion channels and neurotransmitter receptors in the CNS. A shows a voltage-gated channel in which a voltage sensor component of the protein controls the gating (broken arrow) of the channel. B shows a ligand-gated channel in which the binding of the neurotransmitter to the ionotropic channel receptor controls the gating (broken arrow) of the channel. C shows a G protein-coupled (metabotropic) receptor, which, when bound, activates a heterotrimeric G protein. D and E show two ways metabotropic receptors can regulate ion channels. The activated G protein can interact directly to modulate an ion channel (D) or the G protein can activate an enzyme that generates a diffusible second messenger (E), eg, cAMP, which can interact with the ion channel or can activate a kinase that phosphorylates and modulates a channel.
Neurotransmitters exert their effects on neurons by binding to two distinct classes of receptor. The first class is referred to as ligand-gated channels, or ionotropic receptors. These receptors consist of multiple subunits, and binding of the neurotransmitter ligand directly opens the channel, which is an integral part of the receptor complex (see Figure 22–6). These channels are insensitive or only weakly sensitive to membrane potential. Activation of these channels typically results in a brief (a few milliseconds to tens of milliseconds) opening of the channel. Ligand-gated channels are responsible for fast synaptic transmission typical of hierarchical pathways in the CNS (see following text).
Evolution is tireless in the development of natural toxins. A vast number of variations are possible with even a small number of amino acids in peptides, and peptides make up only one of a broad array of toxic compounds. For example, the predatory marine snail genus Conus includes over 3000 different species. Each species kills or paralyzes its prey with a venom that contains 50–200 different peptides or proteins. Furthermore, there is little duplication of peptides among Conus species. Other animals with useful toxins include snakes, frogs, spiders, bees, wasps, and scorpions. Plant species with toxic (or therapeutic) substances are referred to in several other chapters of this book.
Since many toxins act on ion channels, they provide a wealth of chemical tools for studying the function of these channels. In fact, much of our current understanding of the properties of ion channels comes from studies utilizing only a small percentage of the highly potent and selective toxins that are now available. The toxins typically target voltage-sensitive ion channels, but a number of very useful toxins block ionotropic neurotransmitter receptors. Table 21–1 lists some of the toxins most commonly used in research, their mode of action, and their source.
The second class of neurotransmitter receptor is referred to as metabotropic receptors (Figure 21–2C). These are seven-transmembrane G protein-coupled receptors of the type described in Chapter 2. The binding of neurotransmitter to this type of receptor does not result in the direct gating of a channel. Rather, binding to the receptor engages a G protein, which results in the production of second messengers that mediate intracellular signaling cascades such as those described in Chapter 2.
In neurons, activation of metabotropic neurotransmitter receptors often leads to the modulation of voltage-gated channels. These interactions can occur entirely within the plane of the membrane and are referred to as membrane-delimited pathways (Figure 21–2D). In this case, the G protein (often the βγ subunit) interacts directly with a voltage-gated ion channel. In general, two types of voltage-gated ion channels are the targets of this type of signaling: calcium channels and potassium channels. When G proteins interact with calcium channels, they inhibit channel function. This mechanism accounts for the inhibition of neurotransmitter release that occurs when presynaptic metabotropic receptors are activated. In contrast, when these receptors are postsynaptic, they activate (cause the opening of) potassium channels, resulting in a slow postsynaptic inhibition. Metabotropic receptors can also modulate voltage-gated channels less directly by the generation of diffusible second messengers (Figure 21–2E). A classic example of this type of action is provided by the β adrenoceptor, which generates cAMP via the activation of adenylyl cyclase (see Chapter 2). Whereas membrane-delimited actions occur within microdomains in the membrane, second messenger-mediated effects can occur over considerable distances. Finally, an important consequence of the involvement of G proteins in receptor signaling is that, in contrast to the brief effect of ionotropic receptors, the effects of metabotropic receptor activation can last tens of seconds to minutes. Metabotropic receptors predominate in the diffuse neuronal systems in the CNS (see below).
THE SYNAPSE & SYNAPTIC POTENTIALS
The communication between neurons in the CNS occurs through chemical synapses in the majority of cases. (A few instances of electrical coupling between neurons have been documented, and such coupling may play a role in synchronizing neuronal discharge. However, it is unlikely that these electrical synapses are an important site of drug action.) The events involved in synaptic transmission can be summarized as follows.
An action potential propagating down the axon of the presynaptic neuron enters the synaptic terminal and activates voltage-sensitive calcium channels in the membrane of the terminal (see Figure 6–3). The calcium channels responsible for the release of neurotransmitter are generally resistant to the calcium channel-blocking agents discussed in Chapter 12 (verapamil, etc) but are sensitive to blockade by certain marine toxins and metal ions (see Tables 21–1 and 12–4). As calcium flows into the terminal, the increase in intraterminal calcium concentration promotes the fusion of synaptic vesicles with the presynaptic membrane. The neurotransmitter contained in the vesicles is released into the synaptic cleft and diffuses to the receptors on the postsynaptic membrane. The neurotransmitter binds to its receptor and opens channels (either directly or indirectly as described above) causing a brief change in membrane conductance (permeability to ions) of the postsynaptic cell. The time delay from the arrival of the presynaptic action potential to the onset of the postsynaptic response is approximately 0.5 ms. Most of this delay is consumed by the release process, particularly the time required for calcium channels to open.
FIGURE 21–3 Postsynaptic potentials and action potential generation. A (top) shows the voltage recorded upon entry of a microelectrode into a postsynaptic cell and subsequent recording of a resting membrane potential of −60 mV. Stimulation of an excitatory pathway (E1, left) generates transient depolarization called an excitatory postsynaptic potential (EPSP). Simultaneous activation of multiple excitatory synapses (E1 + E2, middle) increases the size of the depolarization, so that the threshold for action potential generation is reached. Alternatively, a train of stimuli from a single input can temporally summate to reach the threshold (E1 + E1, right). B (bottom) demonstrates the interaction of excitatory and inhibitory synapses. On the left, a suprathreshold excitatory stimulus (E3) evokes an action potential. In the center, an inhibitory pathway (I) generates a small hyperpolarizing current called an inhibitory postsynaptic potential (IPSP). On the right, if the previously suprathreshold excitatory input (E3) is given shortly after the inhibitory input (I), the IPSP prevents the excitatory potential from reaching threshold.
The first systematic analysis of synaptic potentials in the CNS was in the early 1950s by Eccles and associates, who recorded intracellularly from spinal motor neurons. When a microelectrode enters a cell, there is a sudden change in the potential recorded by the electrode, which is typically about −60 mV (Figure 21-3). This is the resting membrane potential of the neuron. Two types of pathways—excitatory and inhibitory—impinge on the motor neuron.
When an excitatory pathway is stimulated, a small depolarization or excitatory postsynaptic potential (EPSP) is recorded. This potential is due to the excitatory transmitter acting on an ionotropic receptor, causing an increase in cation permeability. As additional excitatory synapses are activated, there is a graded summation of the EPSPs to increase the size of the depolarization (Figure 21–3
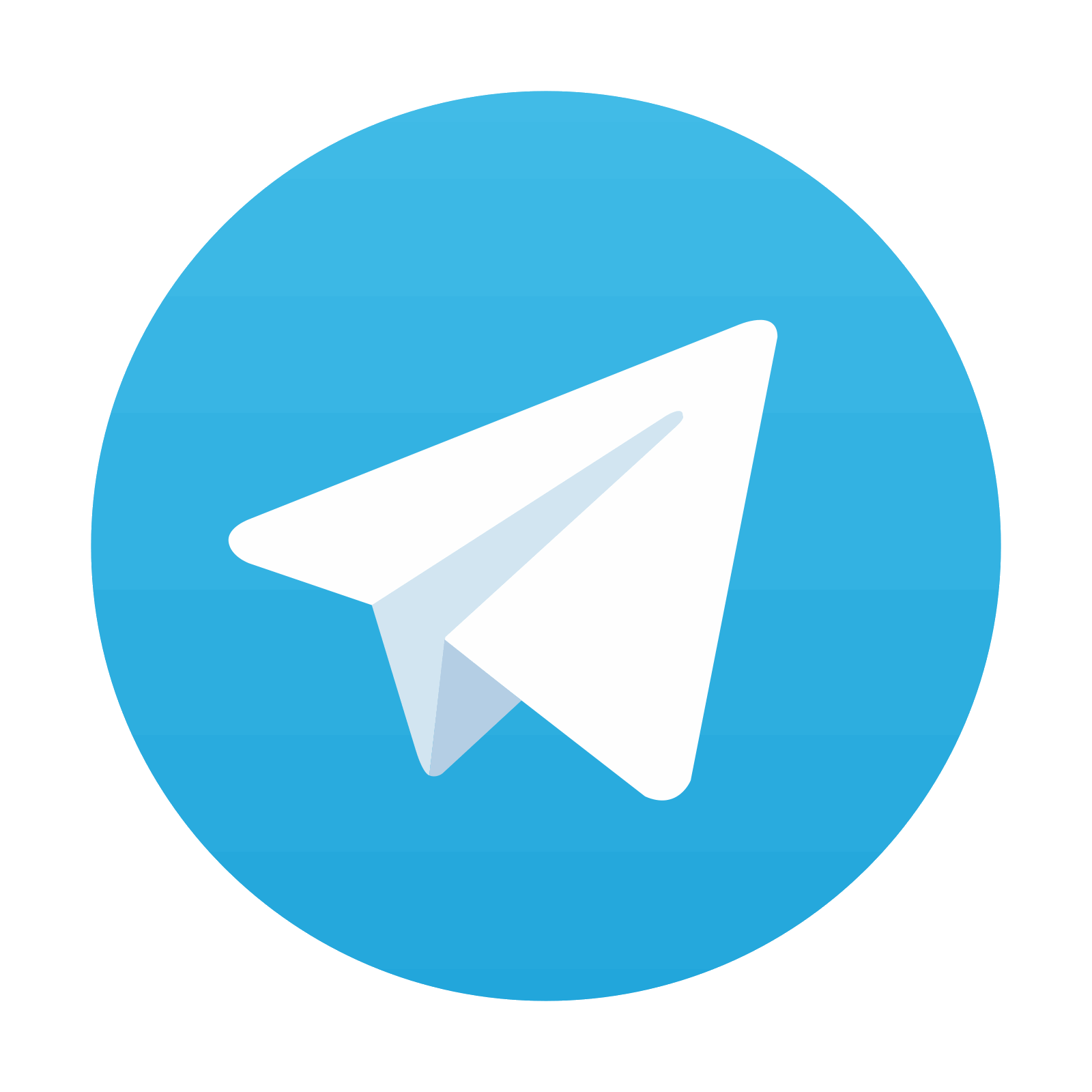
Stay updated, free articles. Join our Telegram channel
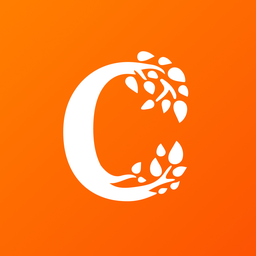
Full access? Get Clinical Tree
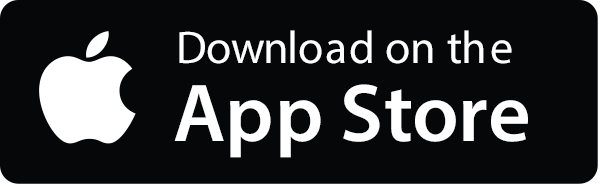
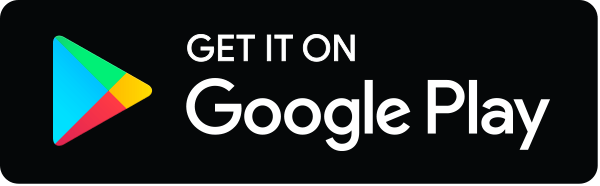