Chapter 2 Water, sodium and potassium
Introduction
Water distribution
Water accounts for approximately 60% of body weight in men and 55% in women, the difference reflecting the typically greater body fat content in women. Approximately 66% of this water is in the intracellular fluid (ICF) and 33% in the extracellular fluid (ECF); only 8% of body water is in the plasma (Fig. 2.1). Water is not actively transported in the body. It is, in general, freely permeable through the ICF and ECF and its distribution is determined by the osmotic contents of these compartments. Except in the kidneys, the osmotic concentrations, or osmolalities, of these compartments are always equal: they are isotonic. Any change in the solute content of a compartment engenders a shift of water, which restores isotonicity.
The major contributors to the osmolality of the ECF are sodium and its associated anions, mainly chloride and bicarbonate; in the ICF the predominant cation is potassium. Other determinants of ECF osmolality include glucose and urea. Protein makes a numerically small contribution of approximately 0.5%. This is because osmolality is dependent on the molar concentrations of solutes: although the total concentration of plasma proteins is approximately 70 g/L, their high molecular weight results in their combined molar concentrations being <1 mmol/L. However, as the capillary endothelium is relatively impermeable to protein and as the protein concentration of interstitial fluid is much less than that of plasma, the osmotic effect of proteins is an important factor in determining water distribution between these two compartments. The contribution of proteins to the osmotic pressure of plasma is known as the ‘colloid osmotic pressure’ or ‘oncotic pressure’ (see Chapter 13).
Under normal circumstances, the amounts of water taken into the body and lost from it are equal over a period of time. Water is obtained from the diet and oxidative metabolism and is lost through the kidneys, skin, lungs and gut (Fig. 2.2). Some 170 L of water is filtered by the kidneys every 24 h, and almost all of this is reabsorbed. The minimum volume of urine necessary for normal excretion of waste products is about 500 mL/24 h but, as a result of obligatory losses by other routes, the minimum daily water intake necessary for the maintenance of water balance is approximately 1100 mL. This increases if losses are abnormally large, for example with excessive sweating or diarrhoea. Water intake is usually considerably greater than this minimum requirement, but the excess is easily excreted through the kidneys.
Potassium distribution
Potassium is the predominant intracellular cation. Some 90% of the total body potassium is free and therefore exchangeable, while the remainder is bound in red blood cells, bone and brain tissue. However, only approximately 2% (50–60 mmol) of the total is located in the extracellular compartment (see Fig. 2.1), where it is readily accessible for measurement. Plasma potassium concentration is not, therefore, an accurate index of total body potassium status, but, because of the effect of potassium on membrane excitability, is important in its own right. The potassium concentration of serum is 0.2–0.3 mmol/L higher than that of plasma, owing to the release of potassium from platelets during clot formation, but this difference is not usually of practical significance.
Water and sodium homoeostasis
Water and ECF osmolality
Changes in body water content independent of the amount of solute will alter the osmolality (Fig. 2.3). The osmolality of the ECF is normally maintained in the range 282–295 mmol/kg of water. Any loss of water from the ECF, such as occurs with water deprivation, will increase its osmolality and result in movement of water from the ICF to the ECF. However, a slight increase in ECF osmolality will still occur, stimulating the hypothalamic thirst centre, causing thirst and thus promoting a desire to drink, and stimulation of the hypothalamic osmoreceptors, which causes the release of vasopressin (antidiuretic hormone, ADH).
Vasopressin renders the renal collecting ducts permeable to water (its combination with V2 receptors results in the insertion of aquaporins (water channels) into the normally impermeable apical membrane of the cells of the collecting tubules), permitting water reabsorption and concentration of the urine; the maximum urine concentration that can be achieved in humans is about 1200 mmol/kg. The osmoreceptors are highly sensitive to osmolality, responding to a change of as little as 1%. Plasma vasopressin concentration falls to very low values at an osmolality of 282 mmol/kg, but rises sharply if osmolality increases above this level (Fig. 2.4A). However, if an increase in ECF osmolality occurs as a result of the presence of a solute such as urea that diffuses readily across cell membranes, ICF osmolality also increases and osmoreceptors are not stimulated.
Other stimuli affecting vasopressin secretion (Fig. 2.5) include angiotensin II, arterial and venous baroreceptors and volume receptors (which sense blood pressure and volume, respectively). Hypovolaemia and hypotension increase the slope of the vasopressin response to an increase in osmolality (see Fig. 2.4A) and lower the threshold osmolality for vasopressin secretion. The vasopressin response to a fall in blood pressure is exponential: it is relatively small with small decreases in plasma volume, but greater falls cause a massive increase in vasopressin secretion (se Fig. 2.4B). Osmolar controls are overridden, so that ECF volume is defended (by stimulating water retention) at the expense of a decrease in osmolality.
Sodium and ECF volume
Aldosterone, released from the adrenal cortex in response to activation of the renin–angiotensin system, stimulates sodium reabsorption in the distal parts of the distal convoluted tubules and collecting ducts and is the major factor controlling renal sodium excretion. The control of renin secretion is discussed in detail in Chapter 8, but in essence, renin secretion is stimulated primarily by a decrease in renal perfusion secondary to a decrease in blood volume—specifically a fall in arterial blood volume (effective blood volume, see below).
Natriuretic peptide hormones also have a role in controlling sodium excretion. Atrial natriuretic peptide (ANP) is a 28 amino acid peptide, one of a family of similar peptides, secreted by the cardiac atria in response to atrial stretch following a rise in atrial pressure (e.g. due to ECF volume expansion). ANP acts both directly by inhibiting distal tubular sodium reabsorption and through decreasing renin (and hence aldosterone) secretion. It also antagonizes the pressor effects of norepinephrine (noradrenaline) and angiotensin II (and thus tends to increase GFR) and has a systemic vasodilatory effect. It appears to provide ‘fine tuning’ of sodium homoeostasis but is probably more important in pathological states than physiologically. Two other structurally similar peptides have been identified: one (brain natriuretic peptide, BNP) is secreted by the cardiac ventricles in response to ventricular stretching and has similar properties to ANP; the other (C-type natriuretic peptide, CNP) is present in high concentrations in vascular endothelium and is a vasodilator. Measurement of BNP is of value in the management of patients with suspected cardiac failure (see Chapter 14). Increased secretion of natriuretic peptides has been postulated to be at least in part responsible for the natriuresis seen in cerebral salt-wasting (see p. 27).
In summary, in health, the response to an increase in ECF volume (e.g. as a result of infusing isotonic saline) is a decrease in the secretion of aldosterone (with no change in that of vasopressin), leading to natriuresis. In sodium depletion, an increase in aldosterone secretion leads to sodium retention: free water retention is only stimulated in severe sodium depletion. In general, the control mechanisms for ECF volume respond less rapidly and are less precise than the control mechanisms for ECF osmolality. Unless hypovolaemia is severe, maintenance of osmolality takes precedence. The physiological responses to a decrease in ECF volume are illustrated in Figure 2.6. In addition to the changes in sodium excretion, these also involve changes in the tone of arteriolar smooth muscle, and hence peripheral vascular resistance and blood pressure.
Water and sodium depletion
Water depletion
Water depletion will occur if water intake is inadequate or if losses are excessive. Excessive loss of water without any sodium loss is unusual, except in diabetes insipidus, but, even if there is loss of sodium as well, provided that this is small, the clinical consequences will be related primarily to the water depletion (Fig. 2.7).
Loss of water from the ECF causes an increase in osmolality, which in turn causes movement of water from the ICF to the ECF, thus lessening the increase. Nevertheless, the increase in ECF osmolality will be sufficient to stimulate the thirst centre and vasopressin secretion. Plasma sodium concentration is increased; plasma protein concentration and the haematocrit are usually only slightly elevated. Unless water depletion is due to uncontrolled loss through the kidneys, the urine becomes highly concentrated and there is a rapid decrease in its volume. Because water loss is borne by the total body water pool, and not just the ECF (Fig. 2.8), signs of a reduced ECF volume are not usually present. Furthermore, the increased colloid osmotic pressure of the plasma tends to hold extracellular water in the vascular compartment. Circulatory failure is a very late feature of water depletion: it is much more likely to occur if sodium depletion is also present.
Severe water depletion induces cerebral dehydration, which may cause cerebral haemorrhage through tearing of blood vessels. In the short term, cerebral shrinkage is mitigated somewhat by movement of extracellular ions into cerebral cells, causing an osmotic intracellular shift of water. If dehydration persists, brain cells adapt by synthesizing osmotically active organic compounds (‘osmolytes’), and cerebral oedema may then follow rapid fluid replacement (see Fig. 2.9B).
Sodium depletion
Sodium depletion is seldom due to inadequate oral intake alone, but sometimes inadequate parenteral input is responsible. More often, it is a consequence of excessive sodium loss (Fig. 2.10). Sodium can be lost from the body either isotonically (e.g. in plasma) or hypotonically (e.g. in sweat or dilute urine). In each case, there will be a decrease in ECF volume (see Fig. 2.8), but this will be less if the fluid lost is hypotonic than if it is isotonic, as some of the water loss will be shared with the ICF. The clinical features of sodium depletion (see Fig. 2.10) are primarily a result of the decrease in ECF volume.
The normal responses to hypovolaemia are an increase in aldosterone secretion, stimulating renal sodium reabsorption in the distal convoluted tubules and collecting ducts, and a fall in urine volume as a consequence of a decreased GFR. Significantly increased vasopressin secretion, which stimulates the production of a highly concentrated urine, only occurs with more severe ECF volume depletion (see Fig. 2.4).
The decrease in GFR may lead to pre-renal uraemia (see Case history 4.1). In contrast to the effects of pure water depletion, plasma protein concentration and the haematocrit are usually clearly increased in sodium depletion, unless this is a result of the loss of plasma or blood. Furthermore, because the fluid loss is borne mainly by the ECF, signs of a reduced ECF volume are usually present, and there is a greater risk of peripheral circulatory failure than in water depletion. The features of sodium and water depletion are compared in Figure 2.11.

Figure 2.11 Clinical and laboratory findings in sodium and water depletion. *Unless due to loss of blood.
The plasma sodium concentration can give an indication of the relative amounts of water and sodium that have been lost: plasma sodium will be normal if the fluid lost is isotonic with respect to the ECF and increased if it is hypotonic. With severe sodium depletion, increased vasopressin secretion secondary to the resulting hypovolaemia may cause water retention; plasma volume is then maintained at the expense of osmolality and hyponatraemia develops. Thus the plasma sodium concentration in a sodium-depleted patient may be low, normal or high (Fig. 2.12).
Water and sodium excess
Water excess
This is usually related to an impairment of water excretion (Fig. 2.13). However, the limit to the ability of the healthy kidneys to excrete water is about 20 mL/min and, occasionally, excessive intake is alone sufficient to cause water intoxication. This can sometimes occur in patients with psychiatric disorders. It has also been described in people drinking large amounts of beer with a low solute content, because this results in a low osmotic load for excretion and there is a minimum osmolality below which the urine cannot be diluted further. Increased thirst can occur in organic brain disease (particularly trauma, and following surgery), although decreased thirst is more common. Hyponatraemia is invariably present in water overload. The increased water load is shared by the ICF and ECF.
The clinical features of water overload (see Fig. 2.13) are related to cerebral overhydration; their incidence and severity depend upon the extent of the water excess and its time course. Thus, a patient with a plasma sodium concentration of 120 mmol/L, in whom water retention has occurred gradually over several days, may be asymptomatic, while one in whom this is an acute phenomenon may show signs of severe water intoxication. In the short term, the effects of hypotonicity are mitigated to some extent by a movement of ions out of cerebral glial cells; more chronically (days), a decrease in intracellular organic ‘osmolytes’ further reduces intracellular water content (see Fig 2.9A). As is the case with water depletion, this adaptation necessitates a cautious approach to treatment, particularly in chronic water overload. The management of water overload is discussed together with that of hyponatraemia on p. 28.
Sodium excess
Sodium excess can result from increased intake or decreased excretion. The clinical features are related primarily to expansion of ECF volume (Fig. 2.14). When related to excessive intake (e.g. the inappropriate use of hypertonic saline), a rapid shift of water from the intracellular compartment may also cause cerebral dehydration. When sodium overload is due to excessive intake, hypernatraemia is usual (see Case history 2.5).
Sodium overload is more usually due to impaired excretion than to excessive intake. The most frequent cause is secondary aldosteronism. This is seen in patients who, despite clinical evidence of increased ECF volume (e.g. peripheral oedema), appear to have a decreased effective arterial blood volume, due, for example, to venous pooling or a disturbance in the normal distribution of ECF between the vascular and extravascular compartments. This phenomenon is particularly associated with cardiac failure, hypoalbuminaemia and hepatic cirrhosis. Many such patients with sodium excess are, paradoxically, hyponatraemic, implying the coexistence of a defect in free water excretion. This is probably in part due to an increase in vasopressin secretion as a result of the decreased effective blood volume. Also, the decrease in GFR and consequent increase in proximal tubular sodium reabsorption decreases the delivery of sodium and chloride to the loops of Henle and distal convoluted tubules. This reduces the diluting capacity of the kidneys, thereby compromising water excretion. Renal disease is a relatively uncommon cause of sodium excess, as is increased mineralocorticoid secretion due to primary adrenal disease (as in Conn’s syndrome, see p. 147).
Laboratory assessment of water and sodium status
• patients with dehydration or excessive fluid loss, as a guide to appropriate replacement
• patients on parenteral fluid replacement who are unable to indicate or respond to thirst (e.g. the comatose, infants and the elderly)
• patients with unexplained confusion, abnormal behaviour or signs of CNS irritability.
Analysis of urine can provide valuable information, but results may be misleading. It should be established whether the urine volume and composition are physiologically appropriate for the patient’s water and sodium status. If they are not, the reason should be sought. For example, a low urinary sodium excretion is to be expected in a patient with hyponatraemia who is sodium depleted (unless this is due to renal sodium loss: natriuresis in such a patient would imply either a failure of aldosterone secretion or a failure of the kidney to respond to the hormone (Case history 2.1)).
Sodium measurement
Under most circumstances, the two techniques give results that are, for practical clinical purposes, the same. However, as activity is a measure of sodium in the water fraction of plasma (normally 93% by volume), significant discrepancies between activity and concentration may arise if the fractional plasma water content is decreased, such as in severe hyperlipidaemia or hyperproteinaemia. The sodium concentration, measured by flame photometry in mmol/L of plasma, will be less than the concentration inferred from the activity. This is because, although the concentration of sodium in plasma water is unchanged, there is less water and thus less sodium in a given volume of plasma. Analysers employing electrodes for which the plasma is diluted before measurement also give a spuriously low result. This effect, known as pseudohyponatraemia, is only seen with severe hyperlipidaemia (when the plasma will usually appear turbid to the naked eye) (see Case history 14.2) and with large increases in total protein due to paraproteinaemia. If it is suspected, plasma osmolality should be measured: it is osmolality that is regulated by the hypothalamus through the release of vasopressin. Plasma osmolality should be normal in a patient with pseudohyponatraemia.
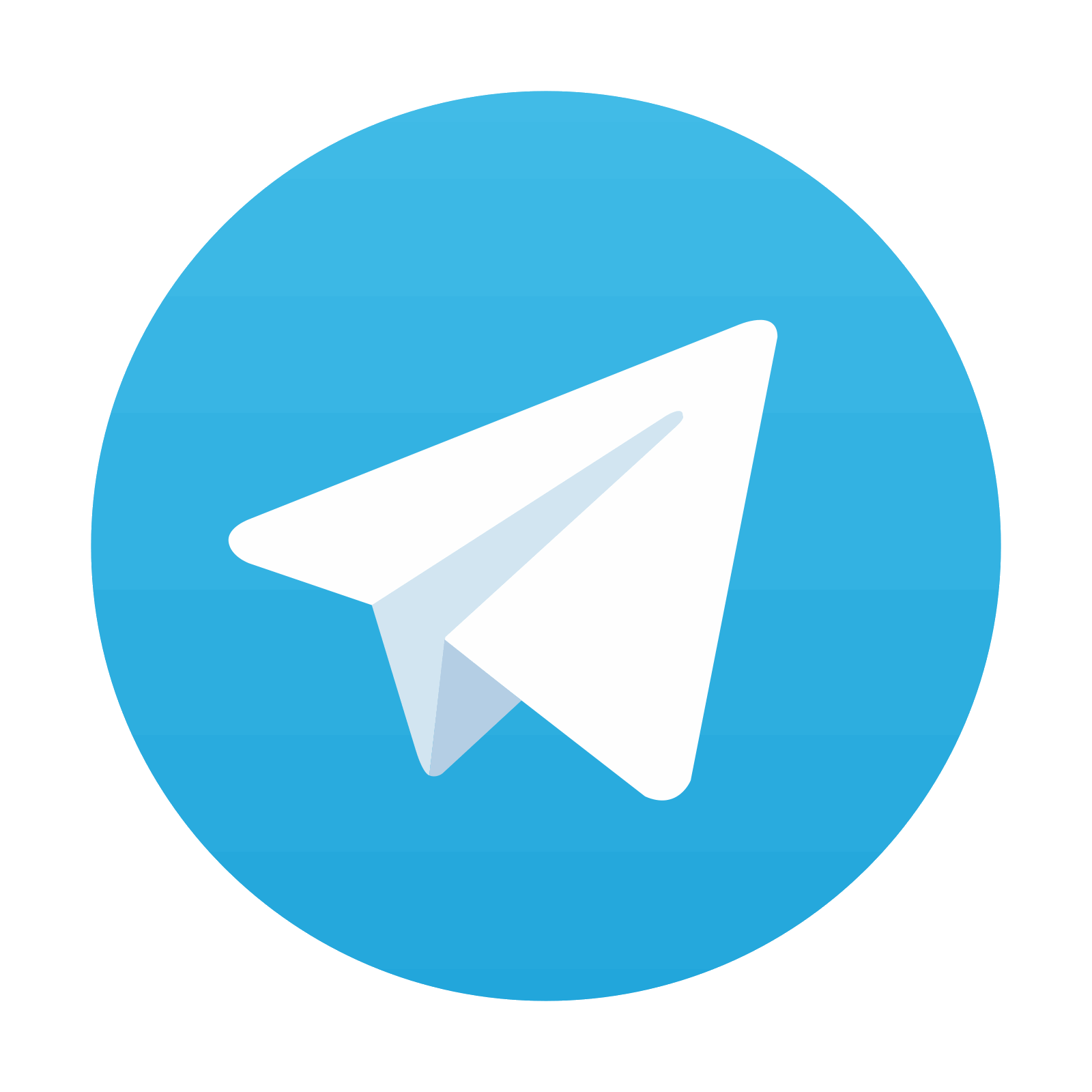
Stay updated, free articles. Join our Telegram channel
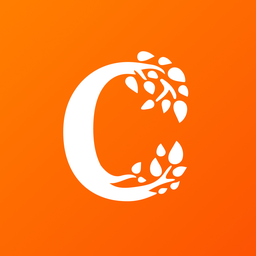
Full access? Get Clinical Tree
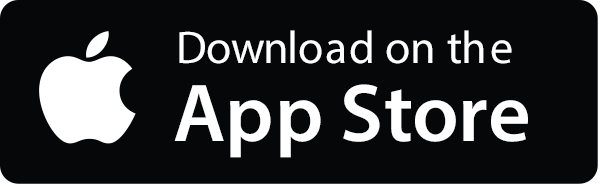
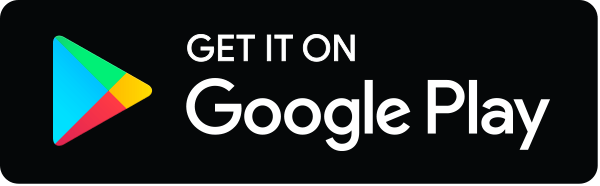