Vitamin B61
Vanessa R. Da Silva
Amy D. Mackey
Steven R. Davis
Jesse F. Gregory III
1Abbreviations: 4-PA, 4-pyridoxic acid; ALAS, δ-aminolevulinate synthase; CRP, C-reactive protein; FAD, flavin adenine dinucleotide; FMN, flavin mononucleotide; HPLC, high-performance liquid chromatography; NAD, nicotinamide adenine dinucleotide; PL, pyridoxal; PLP, pyridoxal 5′-phosphate; PM, pyridoxamine; PMP, pyridoxamine 5′-phosphate; PN, pyridoxine; PNG, pyridoxine 5′-β-D-glucoside; PNP, pyridoxine 5′-phosphate; RDA, recommended dietary allowance; SHMT, serine hydroxymethyltransferase; TNAP, tissue-nonspecific phosphatase; VD, vascular disease.
Since the 1930s, when evidence of vitamin B6 was first reported, our understanding of its properties, metabolic function, and role in maintaining health has expanded enormously. In spite of these advances, areas of uncertainty still exist, including the optimal intake of the vitamin, the consequences of inadequacy, the best way to assess nutritional status, and the effects of supplementation on health. Since the previous edition of this book (1), important advances have occurred regarding the role of vitamin B6 nutritional status and the incidence of chronic disease, and this topic is given special consideration here.
HISTORY
Evidence of a water-soluble nutritional factor later identified as vitamin B6 was first reported in 1934 (2). Five laboratories reported the independent isolation and crystallization of pyridoxine (PN) in 1938 (3, 4, 5, 6, 7), and the proposed structure was confirmed through successful synthesis the following year. Studies of the nutritional requirements of lactic acid bacteria led to the recognition of pyridoxal (PL) and pyridoxamine (PM) (8). The coenzyme form of vitamin B6 was demonstrated to be a phosphorylated derivative (9), and eventually it was identified as the 5′-phosphate.
CHEMISTRY AND NOMENCLATURE
The term vitamin B6 is the preferred generic descriptor (10) for the family of 2-methyl,3-hydroxy,5-hydroxymethylpyridine derivatives that exhibit the nutritional activity of PN. “Pyridoxine” has been used as a generic term especially in clinical contexts; however, it is strongly recommended that the consistent use of the generic “vitamin B6” rather than “pyridoxine” be adopted to reduce confusion in vitamin B6 nomenclature.
Vitamin B6 exists as three main derivatives of a 2-methyl,3-hydroxy,5-hydroxymethylpyridine nucleus that differ with respect to their substituent at the 4 position of the pyridine ring (Fig. 24.1). For the C-4 substituent, PN has a hydroxymethyl group, PL is an aldehyde, whereas PM has an aminomethyl group. Because PN is an alcohol, PN has been termed “pyridoxol” intermittently; this designator is obsolete and should be discontinued. PN, PL, and PM all can exist with a phosphate group esterified at the C-5′ position (i.e., PNP, PLP, and PMP). Pyridoxal 5′-phosphate (PLP) and pyridoxamine 5′-phosphate (PMP) are the coenzyme forms of vitamin B6 and are interconverted as they function in the actions of the aminotransferase family of enzymes. Although pyridoxine 5′-phosphate (PNP) is not a coenzyme, it is an important intermediate in the metabolic pathway by which PLP is formed from dietary PN (Fig. 24.2). 4-Pyridoxic acid (4-PA), which is the major metabolically inactive catabolic product of vitamin B6 metabolism, has a carboxyl group at C-4 and is nutritionally and metabolically inactive. Also shown in Figure 24.1 is pyridoxine 5′-β-D-glucoside (PNG), a glycosylated form of vitamin B6 commonly found in foods of plant origin.
ABSORPTION AND BIOAVAILABILITY
Intestinal absorption of vitamin B6 is thought to take place in the jejunum by nonsaturable, passive diffusion of the nonphosphorylated forms (11). However, evidence from in vitro studies using eukaryotic cells suggests that vitamin B6 absorption is pH dependent and exhibits both saturable and nonsaturable components that are concentration dependent (12). The in vitro model of absorption appears to occur through a carrier-mediated pathway that involves proton exchange (12). Dietary PLP, PMP, and PNP are enzymatically dephosphorylated at the brush-border membrane by alkaline phosphatase before absorption (1). Once absorbed, dietary PN, PL, and PM are phosphorylated by pyridoxal kinase for the purpose of metabolic trapping. Addition of the phosphate group at the 5′ position of the pyridine ring creates a negative charge on the molecule that prevents the vitamers from diffusing through the cellular membranes in intestinal mucosal cells and in other tissues. To cross the basolateral membrane and enter into portal circulation, PN, PL, and PM are converted back to a nonphosphorylated form.
The bioavailability of nutrients in foods and supplements is an important issue in evaluating the adequacy of diets and the efficacy of supplements in meeting nutritional requirements and resolving inadequate status. The bioavailability of vitamin B6 in humans consuming a mixed diet is approximately 75% (13), and data from pigs indicate that the digestibility of vitamin B6 from animal sources is approximately 10% greater than from plant sources (14). As has been reviewed (15), vitamin B6 bioavailability is likely to be a function of the degree of entrapment in the food matrix (i.e., nondigestible residue) and the extent of utilization of glycosylated forms of vitamin B6. PNG, the major glycosylated form of vitamin B6 in the human diet, provides an average of approximately 15% of total daily vitamin B6 intake (16), although this percentage varies widely depending on food selection. The bioavailability of purified PNG was only approximately 30% in rats (17, 18) and approximately 50% in humans when compared with free PN (19, 20). Rat (17) and human (19, 20) studies using isotopically labeled PNG found that PNG was effectively absorbed, but it was not completely hydrolyzed in the small intestine to glucose and PN—a metabolically active form of vitamin B6. Intestinal hydrolysis of PNG is catalyzed by two β-glucosidases: a novel, cytoplasmic enzyme designated PNG hydrolase (21); and the brush-border membrane enzyme lactasephlorizin hydrolase (22). PNG also can be absorbed intact,
potentially hydrolyzed by glucosidase activity in the kidney, or excreted unchanged in the urine (19, 20).
potentially hydrolyzed by glucosidase activity in the kidney, or excreted unchanged in the urine (19, 20).
TRANSPORT AND METABOLISM
Vitamin B6, mostly as PL, enters the portal circulation and is bound to albumin in the plasma for transport (23). PL and PLP constitute 75% to 80% of the circulating forms of vitamin B6 (24). Erythrocytes can take up both PN and PL (25); however, erythrocyte vitamin B6 is probably not available for direct uptake from the circulation into tissues.
The liver is the primary site of vitamin B6 metabolism and where PLP is generated for hepatic use and for export to extrahepatic tissues. The phosphorylation of PN, PL, and PM to yield PNP, PLP, and PMP, respectively, is catalyzed by pyridoxal kinase (26). Conversion of PNP and PMP to PLP is catalyzed by the flavin mononucleotide (FMN)-dependent pyridoxamine-(pyridoxine-)5′-phosphate oxidase in the liver (26). This reaction is critical to the metabolism of dietary vitamin B6 because most extrahepatic tissues have comparatively little oxidase activity. Pyridoxamine-(pyridoxine-)5′-phosphate oxidase is subject to strong product inhibition that serves to avoid the production of excessive amounts of PLP (27). Dephosphorylation of PLP and PMP in the liver and other tissues is catalyzed by tissue-nonspecific phosphatase (TNAP) (28) activity as well as by a vitamin B6-specific form of erythrocyte alkaline phosphatase (29). Two hepatic enzymes, riboflavin (flavin adenine dinucleotide [FAD])-dependent aldehyde oxidase and nicotinamide adenine dinucleotide (NAD)-dependent aldehyde dehydrogenase, oxidize excess PL in tissues to 4-PA, the major catabolic product of vitamin B6 (28).
As stated earlier, PLP and PL are the predominant circulating forms of vitamin B6. The turnover of vitamin B6, as PLP, has been described using a compartmental model that includes five body pools: muscle, liver, plasma, erythrocytes, and one to combine all other pools (30). Total body concentrations of vitamin B6 are estimated to be 15 nmol/g, which corresponds to approximately 1000 µmol in an adult human (31). PLP in the muscle pool represents 75% to 80% of total body vitamin B6 and serves as a coenzyme for glycogen phosphorylase (31).
Tissue uptake of vitamin B6 from circulation requires dephosphorylation. After enzymatic removal of the 5′-phosphate group by plasma membrane TNAP, vitamin B6 can cross cellular membranes by a carrier-mediated transport system (12, 32). Vitamin B6 in tissues is retained by phosphorylation and concentrated to the mitochondria and cytoplasm.
FUNCTIONS
Vitamin B6 functions as a coenzyme in various enzymatic reactions in the metabolism of amino acids, one-carbon units, lipids, and the pathways of gluconeogenesis, heme, and neurotransmitter biosynthesis. PLP is the most common vitamin B6 coenzyme. The structures of PLP and PMP and their respective nonphosphorylated forms accommodate the formation of Schiff base linkages with other amines (PL/PLP) and aldehydes (PM/PMP). The structures of these vitamers make them well suited to serve as coenzymes for more than 100 different enzymes.
Amino Acids
Nearly all amino acids require at least one PLP-dependent enzyme in their metabolism. PLP is a coenzyme for aminotransaminases that catalyze reversible conversions of amino acids to their corresponding α-keto acids with simultaneous transfer of the amino group to yield PMP. Amino acids can also be modified by PLP-dependent decarboxylation and desulfuration reactions. Metabolism of several amino acids involved in one-carbon metabolism is catalyzed by PLP-dependent reactions, as described later. PLP-dependent decarboxylation reactions are important in the biosynthesis of neurotransmitters (γ-aminobutyric acid, dopamine, and norepinephrine), including the conversion of L-aromatic amino acids to active neurotransmitters (e.g., tryptophan conversion to serotonin) (33).
One-Carbon Units
PLP is a coenzyme for four enzymes in one-carbon metabolism and transsulfuration. Serine hydroxymethyltransferase (SHMT) and glycine decarboxylase transfer one-carbon units to tetrahydrofolate from serine and glycine, respectively (Fig. 24.3, reactions 1 and 2). These enzymatic reactions provide most of the onecarbon groups used for purine and thymidine synthesis, as well as the methyl groups for remethylation of homocysteine to methionine (34, 35). The methyl groups incorporated into methionine may be used for S-adenosylmethionine-dependent transmethylation reactions involved in the metabolism of creatine, DNA, RNA, lipids, proteins, and other molecules. The transsulfuration pathway is composed of the PLP-dependent enzymes cystathionine β-synthase and cystathionine γ-lyase (see Fig. 24.3, reactions 3 and 4). In this pathway, homocysteine is condensed with serine to produce cystathionine, which is subsequently cleaved to produce cysteine and α-aminobutyrate. Transsulfuration and generation of one-carbon units are impaired in severely vitamin B6-deficient rats (36, 37). Elevated concentrations of glycine and cystathionine in plasma from individuals with low vitamin B6 status suggest similar effects in humans during moderate vitamin B6 restriction. Cysteine concentration in plasma and red blood cells and the rate of generation of one-carbon units were not significantly affected by marginal vitamin B6 status (20 to 30 nmol PLP/L) in healthy young adults, however (38, 39, 40, 41). The level of vitamin B6 deficiency that
is required to affect the function of these pathways in humans remains unclear.
is required to affect the function of these pathways in humans remains unclear.
![]() Fig. 24.3. Pyridoxal-5′-phosphate (PLP) dependence of homocysteine (Hcy) and other one-carbon cycle reactions: (1) serine (Ser) hydroxymethyltransferase; (2) glycine (Gly) decarboxylase of the glycine cleavage system; (3) cystathionine (Csn) β-synthase; (4) cystathione γ-lyase. CH2THF, 5,10-methylenetetrahydrofolate; CH3THF, 5-methyltetrahydrofolate; Cys, cysteine; Met, methionine; RM, remethylation; SAH, S-adenosylhomocysteine; SAM, S-adenosylmethionine; TM, transmethylation; TS, transsulfuration. |
Lipids
The role of vitamin B6 in lipid metabolism is not clearly defined. Vitamin B6-deficient rats had altered fatty acid profiles of tissue lipids, with reduced concentrations of arachidonic acid and increased linoleic acid (42), whereas plasma cholesterol and triglyceride concentrations were increased (42). The biochemical mechanisms involved are uncertain; however, these observations may be explained by aberrations in the PLP-dependent enzymatic pathways involved in the transfer of methyl groups, which could yield lower concentrations of methylated phospholipids. The biosynthetic pathway of carnitine, which is essential for intramitochondrial transport of longchain fatty acids, requires the activity of PLP-dependent 3-hydroxytrimethyl-lysine aldolase. Vitamin B6 deficiency was shown to reduce plasma carnitine concentrations in rats (43) but not in humans (1).
Glycogenolysis and Gluconeogenesis
Vitamin B6, as PLP, plays a dual role in the synthesis of glucose. Glycogen phosphorylase relies on PLP as a coenzyme in the enzymatic cleavage of glycogen that sequentially releases glucose-1-phosphate units. The 5′-phosphate group of PLP, rather than the 4′-formyl group (as in the aminotransferase reactions), is required for general acid catalysis in glycogenolysis. Both glycogen phosphorylase activity and muscle concentrations of vitamin B6 are resilient to effects of dietary B6 restriction (44, 45). PLP-dependent transaminases convert gluconeogenic amino acids to α-keto acids to create substrates for the production of glucose. The effect of vitamin B6 status on endogenous gluconeogenesis remains unclear.
Heme Biosynthesis
Heme biosynthesis relies on the activity of PLP-dependent δ-aminolevulinate synthase (ALAS). This enzyme catalyzes the condensation of succinyl coenzyme A and glycine to form δ-aminolevulinate, which is the precursor for the porphyrin ring. Chronic vitamin B6 deficiency can precipitate microcytic hypochromic anemia in which the hemoglobin concentration of erythrocytes is reduced. Sideroblastic anemia is an inherited form of ALAS deficiency. This type of anemia often can be successfully treated with PN supplementation; however, some mutations alter the PLP binding sites on the ALAS enzyme (46) and thus diminish the efficacy of PN supplementation.
Interactions with Other Nutrients
The interconversion and metabolism of vitamin B6 depend on riboflavin, niacin, and zinc (see Fig. 24.2). Both PN (PM) phosphate oxidase and aldehyde oxidase require riboflavin in the forms of FMN and FAD, respectively. Niacin, as NAD, serves as coenzyme for aldehyde dehydrogenase. The phosphorylation of vitamin B6 is catalyzed by PL kinase, which requires zinc as cofactor. Insufficient dietary intake of these nutrients may adversely affect the metabolic utilization of vitamin B6.
Niacin, folate, and carnitine require vitamin B6 for their biosynthesis and metabolism. Biosynthesis of niacin from tryptophan is catalyzed by PLP-dependent kynureninase. As discussed earlier, PLP-dependent SHMT and glycine decarboxylase are essential for normal folate metabolism (see Fig. 24.3, reactions 1 and 2). Carnitine is synthesized from lysine and methionine in a multistep process that requires PLP.
VITAMIN B6 IN FOODS AND SUPPLEMENTS
Vitamin B6 traditionally has been measured in foods and biologic materials by microbiologic assays using the yeast Saccharomyces uvarum (47). Both microbiologic and high-performance liquid chromatographic (HPLC) methods are now widely used (47). Microbiologic assays are best suited to the measurement of total vitamin B6, whereas properly configured HPLC methods allow the determination of the various forms of vitamin B6, including the glycosylated form(s) (48).
Vitamin B6 is widely distributed throughout the food supply. Foods of animal origin such as meat, fish, eggs, and dairy products are rich in vitamin B6, mostly as PL and PM and their phosphorylated forms. Many vegetables and whole grain cereal products are good sources of the vitamin. The predominant forms of vitamin B6 in foods of plant origin are PN and glycosylated PN (11). Plant tissues may contain up to 75% of their vitamin B6 as PNG (49), which is presumed to be a storage form of the vitamin. According to the Continuing Survey of Food Intakes by Individuals in 1995 (unpublished data), the adult US population obtains most of its dietary vitamin B6 from
ready-to-eat fortified cereals, meat, fish, poultry, starchy vegetables, and noncitrus fruits.
ready-to-eat fortified cereals, meat, fish, poultry, starchy vegetables, and noncitrus fruits.
Relatively little loss of vitamin B6 occurs during food storage and handling, aside from losses occurring during the milling of grain, but losses of PL, PLP, PM, and PMP during cooking and thermal processing of foods can be significant (50). The nutritional impact of vitamin B6 loss was acutely observed in the 1950s when infants were fed formula for which a change in thermal processing conditions led to excessive destruction of vitamin B6 in the unfortified formula (51). Certain infants consuming this formula developed convulsive seizures that were alleviated by PN supplementation. These incidents prompted the routine fortification of infant formulas with PN. Because of its greater stability than the other B6 vitamers, PN hydrochloride is used in all types of food fortification and in most nutritional supplements. PN α-ketoglutarate also has been used as a vitamin B6 supplement to enhance exercise performance; however, the evidence supporting this benefit is equivocal (52).
ASSESSMENT OF NUTRITIONAL STATUS
Vitamin B6 nutritional status can be evaluated using direct measurement of vitamin B6 forms in blood or urine or by indicators based on the biochemical function of vitamin B6 (53, 54). The various indicators of vitamin B6 status and their generally accepted cutoff values are summarized in Table 24.1.
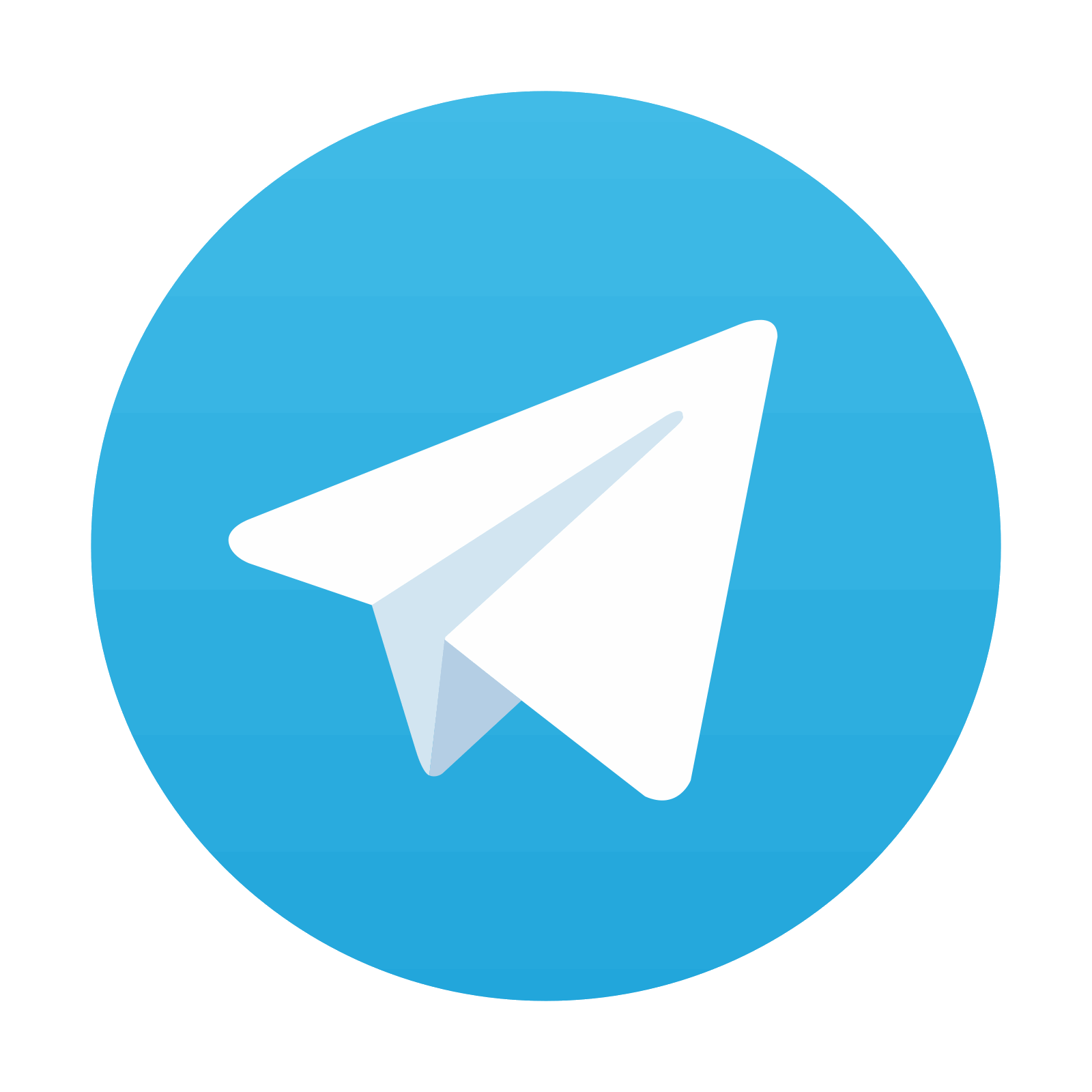
Stay updated, free articles. Join our Telegram channel
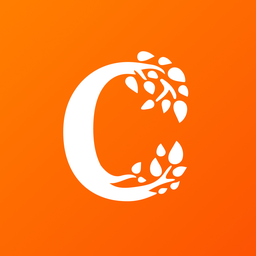
Full access? Get Clinical Tree
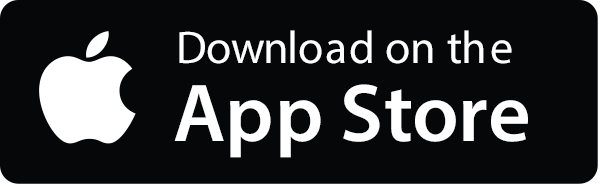
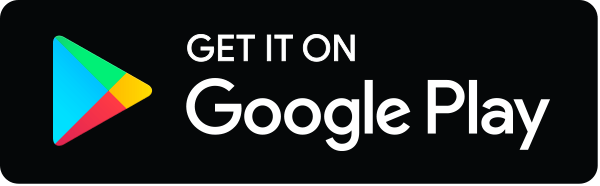