- New blood vessel growth (angiogenesis) plays a central role during the onset and progression of cancer, and the inhibition of vessel growth can therefore be exploited to control tumor growth and metastasis.
- The model of the angiogenic switch postulates that the transition from a normally quiescent blood vessel to a proliferative and invasive endothelial cell sprout during tumor growth is due to an imbalance between angiogenesis stimulators and inhibitors.
- The most potent known stimulators of tumor angiogenesis are the secreted growth factors VEGF and FGF; they cooperate with other signaling molecules such as angiopoietin, members of the EPH and ephrin families, and Notch ligands to control the patterning of vascular networks and vessel structure.
- Endogenous inhibitors of angiogenesis act either locally to modify vessel patterning or systemically to suppress new vessel growth.
- Endostatin and angiostatin are two examples of endogenous inhibitors that are secreted by the primary tumor to suppress tumor metastasis.
- Several endogenous and engineered angiogenesis inhibitors have been approved or are in clinical trials for cancer therapy; amongst these, an antibody-blocking VEGF function has proven beneficial for the treatment of several types of cancer in combination with chemo- and radiotherapy.
Introduction
New blood vessel growth plays a central role during the onset and progression of cancer. Accordingly, inhibiting vessel growth has become an important concept in the design of more efficient cancer therapies. In this chapter, we review our current understanding of the molecular mechanisms that govern vascular growth and differentiation and highlight their significance for tumor expansion. As it is increasingly evident that pathological blood vessel growth reactivates numerous signaling pathways that control tissue vascularization during embryonic and postnatal development, we will relate our current knowledge of vascular development to specific observations derived from studies of tumor vascularization. In addition, we will consider the notion that pathological vascularization depends on additional mechanisms that do not operate during developmental (i.e. physiological) vessel growth, and these mechanisms may affect the success of antiangiogenic therapies.
The vasculature is a flexible conduit that delivers and exchanges nutrients, wastes, hormones, and immune cells. During embryogenesis, blood vessels and the heart are the first recognizable organ system to develop. In the adult, the blood vessel network’s continued maturation and maintenance are critical for tissue metabolism and homeostasis as well as repair processes such as inflammation and wound healing. Excessive vascular growth plays a central role during the onset and progress of a number of adult diseases including cancer and eye diseases.
Blood vessels are composed of two main cellular components, endothelial and mural cells (Fig. 14.1). The endothelium is a continuous, cylindrical epithelial sheet that creates the vessel lumen. The endothelial cells directly interface with blood and are responsible for maintaining a nonthrombogenic surface. A basal lamina separates the endothelium from the second component of vessels, the contractile mural cells. These cells surround the endothelial tube and are primarily responsible for the modulation of vascular tone. In large-caliber vessels such as arteries, veins, arterioles, and venules, the mural cells are called smooth muscle and are organized into multiple cell layers. At the microvasculature level, including capillaries and postcapillary venules, a single discontinuous layer of cells, called pericytes, surrounds the endothelium. Homotypic and heterotypic communication between the endothelium and mural cells contributes to the control of vascular physiology and growth.
Figure 14.1 Blood vessels are composed of endothelial and mural cells. Double-label immunofluorescence reveals that blood vessels are formed from an inner layer of IB4-positive endothelial cells (ec, shown in blue) and an outer layer of NG2-positive pericytes (pc, shown in green). Scale bar: 10 µm.
Image courtesy of Dr Alessandro Fantin
.
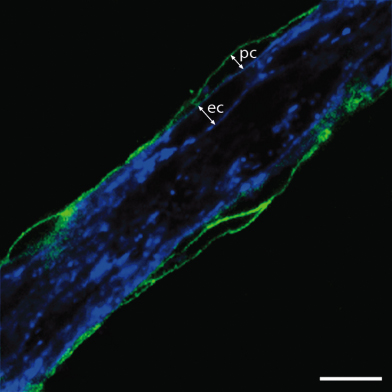
General Principles of New Vessel Growth
In the adult, the vasculature normally represents an extreme example of a stable, quiescent population of cells (see Chapters 4 and 5), with an adult capillary endothelial cell having an estimated turnover time of approximately 1000 days. In contrast, the average turnover for a highly regenerative tissue, such as the gut epithelium, is 2–3 days. The growth of new blood vessels occurs in the adult normally only under the influence of specific physiological stimuli, such as hormonal fluctuations during the female reproductive cycle or chronic increases in tissue metabolism to stimulate new vessel growth.
In contrast to adult life, blood vessel growth occurs rapidly and in almost all tissues of the developing embryo. During embryogenesis, the two well-recognized mechanisms of blood vessel growth are vasculogenesis and angiogenesis (Box 14.1; Fig. 14.2). Vasculogenesis entails the de novo formation of blood vessels from endothelial cell precursors, termed angioblasts, which are derived from the primitive mesenchyme. Vasculogenesis first takes place extra-embryonically in the yolk sac. In the embryo, vasculogenesis is the mode of development for the major axial vessels such as the aorta and for the vascular plexus of some endoderm-derived organs, such as the spleen. Angiogenesis involves the formation of new vessels by sprouting growth from preexisting vessels (Fig. 14.3). This is the main mechanism for vascularization of neural and mesodermal tissues in the embryo, for example the brain and limb bud, and the main mechanism for the neovascularization of adult tissues. Extensive remodeling of blood vessels occurs subsequent or parallel to network formation by angiogenic sprouting, for example to increase vessel caliber or change network properties through vascular intusseception, anastomosis, or selective regression of vessel segments. In addition, remodeling involves the recruitment of mural cells, the acquisition of venous or arterial properties, and an ill-understood increase in vessel stability and resilience that may be linked to changes in basement membrane composition and reciprocal signaling between mural and endothelial cells. Taken together, these mechanisms of vessel maturation appear to make the adult vasculature more refractory to angiogenic activation than is the case for embryonic vessels. In this context, it is interesting to note that some angiogenesis inhibitors regulate vascular growth in the adult, but are not essential for the development of the embryonic vasculature (see “Role of inhibitors in angiogenesis,” this chapter). Finally, endothelial cells within the remodeling blood vessels undergo tissue-specific specializations, for example the acquisition of specialized cell–cell adhesions, termed tight junctions, or transcellular channels, termed fenestrae.
Figure 14.2 Several different classes of secreted molecules cooperate to stimulate vascular growth and differentiation. The life cycle of endothelium may begin with the assembly of angioblasts into a primitive vascular plexus (vasculogenesis) or with the reactivation of quiescent vessel endothelium. Through the process of sprouting growth (angiogenesis), the vessel network begins to expand. New vessels acquire mural cells and adopt an arterial or venous identity; concomitantly, the vascular network continues to branch and remodel into a hierarchical vascular tree. Lastly, vessels specialize according to local physiological needs, for example to form the blood–brain barrier or the fenestrated sieve plates found in the kidney glomerulus. With the notable exception of blood–brain barrier function, most of the processes illustrated are stimulated by VEGF; several other factors act upstream of VEGF or cooperate with VEGF to affect specific aspects of vessel growth and specialization.
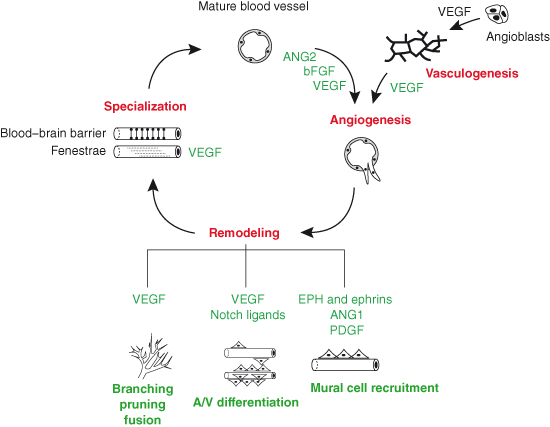
Figure 14.3 Angiogenic sprouting of blood vessels in the developing brain. Isolectin B4-positive blood vessels extend filopodia-studded sprouts to initiate new branch formation during brain vascularization in the midgestation mouse embryo.
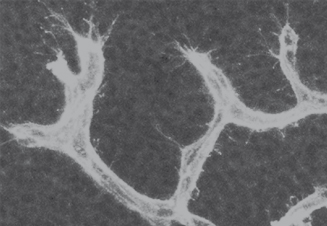
A third mode of vascular growth has been described, which postulates that circulating endothelial progenitors are incorporated into the tumor vasculature (Box 14.1). This mode of vascular growth may bear some mechanistic parallels to embryonic vasculogenesis, but its general significance and mechanisms are not clear as yet. It was originally thought that mobile endothelial progenitors derived from the bone marrow are recruited from the circulation into existing vasculature as a key step in tumor angiogenesis. More recently, bone marrow–derived precursor cells have been studied for their ability to contribute to tumor angiogenesis by adding to the mural complement of the vasculature or by providing transient accessory cells such as monocytes and macrophages, which secrete proangiogenic factors.
Pathological Neovascularization: Tumor Vessels
In stark contrast to physiological neovascular responses, which consist of well-governed bursts of vessel growth limited in both time and space, blood vessel growth that accompanies diseases such as diabetes, psoriasis, and cancer is often characterized by chaotic growth, patterning, and dysfunction. During tumorigenesis, endothelial cell proliferation rapidly increases, with turnover time decreasing from approximately 1000 days in quiescent vessel beds to 50–60 hours. Nascent tumor vessels are also morphologically distinct, as they can be tortuous and of highly variable shape and size; moreover, they often lack a normal complement and juxtaposition of mural cells. In addition to assuming a chaotic structure, the vessels in tumors form disorganized networks bereft of the intricate pattern observed in normal tissues, and there is rarely a clear organization into arterial and venous compartments. Enhanced vascular permeability often accompanies tumor angiogenesis; it contributes to high interstitial pressures in the tumor microenvironment and chaotic blood flow. It has been suggested that both the tortuous nature of vessels and the high interstitial pressure inside the tumor impair delivery of conventional chemotherapeutics into the tumor tissue.
Several different factors likely contribute to the formation of the chaotic tumor vasculature. Firstly, host tissues influence the patterning of growing vessels by providing local cues. This is also observed during physiological angiogenesis, for example during development to control branching morphogenesis, the co-patterning of vessels and nerves, and the positioning and connectivity of the arterio-venous compartments. Such a well-orchestrated elaboration of vessel pattern is most likely not recapitulated during neoplasia, because a disorganized, heterogeneous, and chaotically expanding tumor mass could provide only similarly disorganized angiogenic cues. Secondly, the genetic alterations associated with tumor cell transformation and malignancy may trigger a gross imbalance in the levels of tumor-derived stimulators and inhibitors of angiogenesis (see “Basic concepts in tumor angiogenesis: the angiogenic switch,” this chapter). Finally, an increase in activated immune cells and local tumor-induced changes in the stroma influence tumor vessel morphogenesis and permeability, in part simply by adding even more players to the local cytokine and growth factor “soup.”
Basic Concepts in Tumor Angiogenesis: the Angiogenic Switch
Pathological blood vessel growth occurs in tissues with elevated metabolic demands or reduced blood flow as a means to increase the capacity of the microvasculature. For example, the ischemic retina produces angiogenic factors to stimulate neovascular growth in diabetic retinopathy. In the case of cancer, an increased metabolic load and reduced oxygen provision to the tumor core are believed to be the primary trigger for angiogenesis. The original concept defining the importance of tumor angiogenesis postulates that the expansion of solid tumors beyond 1–2 mm3 is dependent on the growth of new capillaries (Box 14.2). Importantly, the intimate relationship between tumor cells and blood vessels not only promotes the growth of the primary tumor, but also can contribute to metastatic spread. Moreover, neoangiogenesis appears to play a role in the establishment of “liquid tumors” (i.e. hematological malignancies); for example, leukemia is associated with neoangiogenesis in the bone marrow, and leukemic cells can be seen clustered on the new vessels.
A model explaining the transition from a quiescent capillary to a proliferative and invasive endothelial cell sprout during tumorigenesis is based upon the idea that the maintenance of vascular quiescence is due to a precise balance between angiogenesis stimulators and inhibitors. According to this model, a rise in the concentration of stimulators initiates an angiogenic response, whereas the return to quiescence is promoted by a decrease of stimulators. Vice versa, a reduction in the usual level of inhibitors would make endothelial cells more susceptible to stimulatory cues. Such changes in stimulator and inhibitor levels can be induced by a combination of genetic alterations within the tumor cells and physiological demands of the growing tumor mass, which together trigger the “angiogenic switch” of a slowly growing tumor.
This angiogenic switch requires that the tumor either produces its own stimulators of angiogenesis or induces its surrounding cells to secrete such factors. In parallel, tumor cells growing within an avascular environment, such as an epidermal tissue, need to induce the basement membrane’s breakdown to allow invasion of blood vessels. In some instances, for example during metastasis, the switch to an angiogenic phenotype requires that tumor cells exit their host vessel and induce new vessel sprouts from neighboring vessels (co-option). Importantly, the term “switch” does not imply that a specific gene mutation is mandatory – the “switch” may be thrown early as an inevitable accompaniment of the growth-promoting activity of various oncogenes, whose main role may be in driving cellular replication (see the section on RAS and MYC collaboration in cancer in Chapter 6).
The idea that tumor growth and spread are intimately linked to an angiogenic switch, which therefore might be targeted to control cancer, catalyzed an intensive hunt for factors that are elaborated by tumor cells to stimulate angiogenesis. In the wake of this quest, the past three decades of research have shed much light on the complex molecular interplay between the endothelium and its environment during both tumor growth and embryonic development. This effort has given rise to new treatments for cancer that, in turn, validate the antiangiogenesis hypothesis first formalized by Folkman (Box 14.2).
Vascular Growth and Differentiation Factors: Stimulators of the Angiogenic Switch
Numerous stimulators of capillary endothelial cell growth, termed angiogenic factors, have been identified since the search began 30 years ago. Traditionally, assignment of angiogenic activity to biomolecules was dependent on their ability to increase endothelial cell proliferation and/or to stimulate new capillary growth using in vivo
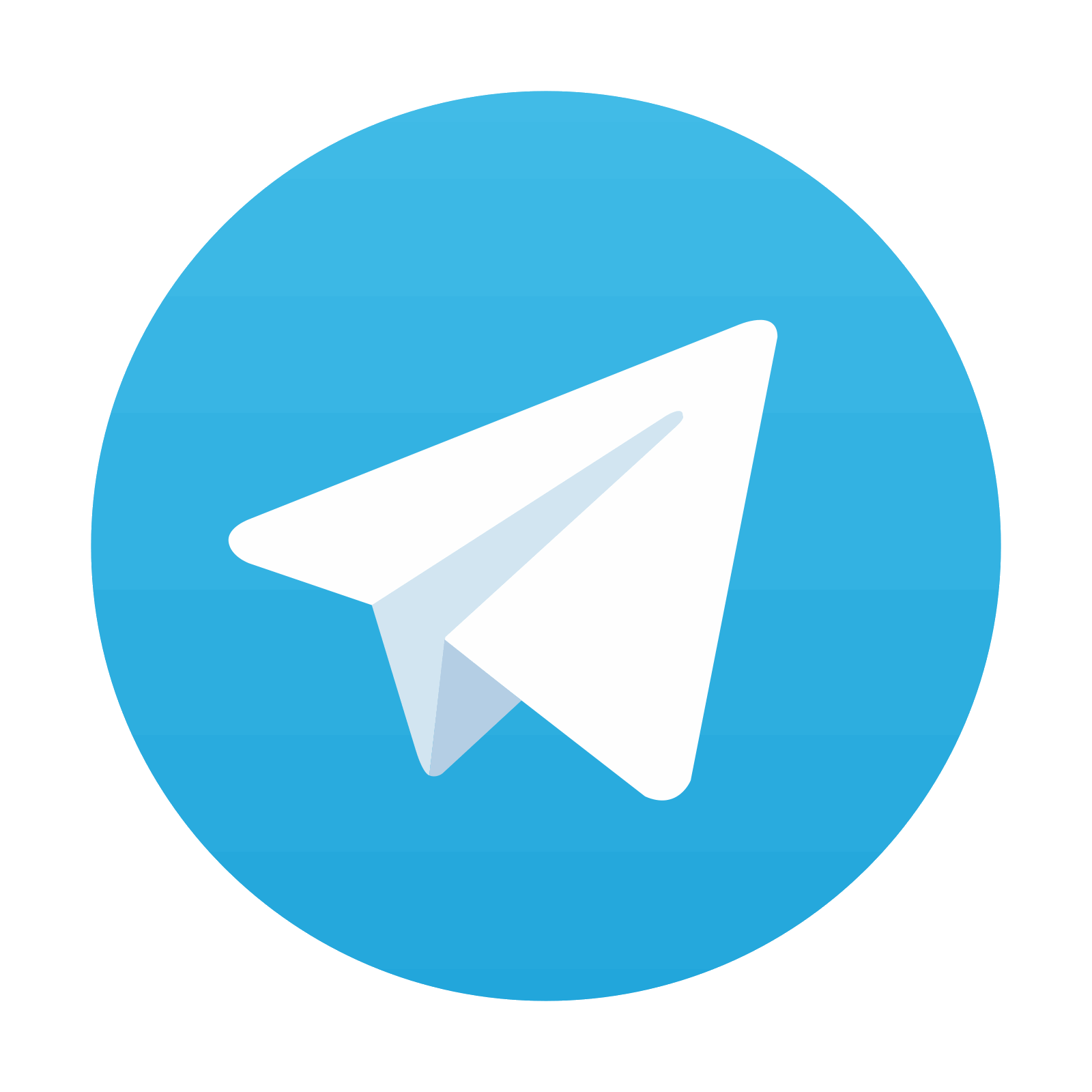
Stay updated, free articles. Join our Telegram channel
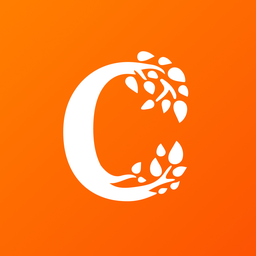
Full access? Get Clinical Tree
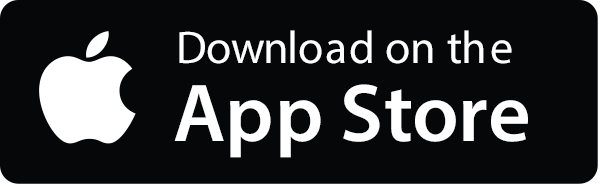
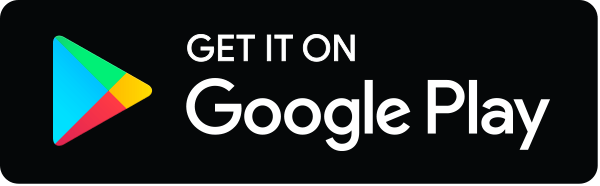