Chapter 19 Koenraad Philippaert*; Rudi Vennekens Katholieke Universiteit of Leuven, Department of Cellular and Molecular Medicine, Laboratory of Ion Channel Research and TRPLe (TRP Research Platform Leuven), Campus Gasthuisberg, Leuven, Belgium Transient receptor potential (TRP) proteins constitute a family of cation channels with diverse permeation and gating properties. Consequently, they have a complex role in mammalian physiology, ranging from temperature sensation in the skin through blood pressure regulation to Ca2 + reabsorption in kidneys and intestines. Increasing evidence has implicated TRP channels in the pathology of diabetes, both on the level of insulin release from the pancreatic ß cells and in secondary conditions such as diabetic neuropathy, nephropathy, and vasculopathy. In this review, we summarize these recent findings. The authors wish to thank all the members of the Laboratory of Ion Channel Research (KU Leuven) and Professor Frans Schuit for stimulating discussions. The FWO Vlaanderen, the Interuniversitary Attraction Poles (IUAP) program of the Belgian federal government and the Bijzonder Onderzoeksfonds Leuven have granted support for the authors’ work. The authors are members of the TRP Research Platform Leuven (TRPLe) consortium Leuven. The transient receptor potential (TRP) family of cation channels consists of 28 mammalian members, which can be subdivided into six main subfamilies based on amino acid homology [1]. Most TRP channels are nonselective Ca2 +-permeable channels, although the permeability ratios PCa/PNa vary considerably [2]. Gating of TRP channels is very diverse [3–7]. TRP channels can be activated by a plethora of stimuli, including ligand binding, voltage, cell swelling, and temperature. As a consequence, they are involved in diverse physiological processes such as heat sensing in sensory nerve endings, taste perception, and the modulation of allergic reactions. Moreover, these channels have been implicated in severe human diseases, such as polycystic kidney disease and mucolipidosis [8,9]. An increasing amount of data points to a role for TRP channels in pancreatic β cells, where they regulate insulin secretion. These data suggest that TRP channels might be interesting drug targets for the treatment of diabetes, and that they could be involved in the development of this important disease. Apart from the pancreatic pathology, diabetes is also associated with neuropathy, vasculopathy, and nephropathy. Considering the wide expression pattern of TRP channels, it is not surprising that TRP channels have also been associated with these aspects of diabetes, and increasing data indicates that their potential as drug targets is even more important in this area as compared to the pancreatic β cell. TRP channels have been described both in primary β cells and insulin-secreting cell lines [10,11]. TRPV2, TRPV4, TRPC1, TRPC4, TRPC6, TRPM2, TRPM3, TRPM4, and TRPM5 are identified in insulinoma cell lines such as MIN6 (mouse) or INS-1 (rat). Expression of TRPV2, TRPV4, TRPC1, TRPM2, TRPM3, TRPM4, and TRPM5 was reported in mouse islets and TRPM2, TRPV5, TRPC1, and TRPC4 in rat islets or β cells. Interestingly, no expression could be found in mouse islets for TRPV5, TRPM1, TRPM8, or TRPP3 (Colsoul, Nilius, & Vennekens, unpublished). Unfortunately, sparse data is available from human tissue: TRPV5 and TRPV6 are detected in human pancreas, and TRPM2, TRPM4, and TRPM5 transcripts are detected in human islets [12–14]. The pancreatic islet is a complex structure consisting of five different cell types: insulin-secreting β cells (which constitute 65-90% of the islet cell population), glucagon-releasing α cells, somatostatin-producing δ cells, polypeptide-containing PP cells, and ghrelin-secreting ε cells. Insulin is synthesized and secreted into the blood by the β cells, mainly in response to glucose but also in response to other nutrients (such as amino acids and fatty acids), hormones (e.g., the incretin hormones GLP-1 and GIP), and neurotransmitters (e.g., ACh) [15,16]. The secretion of insulin by the pancreatic β cell is a complex process driven by electrical activity and oscillations of the intracellular Ca2 + concentration ([Ca2 +]cyt) [17]. Briefly, glucose enters the β cell via the high-affinity glucose transporter (GLUT-2), and glucose metabolism increases intracellular ATP levels, which closes ATP-sensitive K+ channels. This increases the input resistance of the β cell, allowing a small inward current, whose molecular identity is still elusive, to generate a significant depolarization [18–20]. Notably, TRP channels were often hypothesized to be interesting candidates for this background depolarizing current [11,21]. What follows is a typical pattern of bursts of action potentials from a depolarized plateau and parallel oscillatory increases of [Ca2 +]cyt. This pattern results from a complex interplay between ATP-sensitive K+ channels, voltage-dependent Ca2 + and K+ channels, and the cellular metabolism of the β cell [21–23]. Increased [Ca2 +]cyt triggers exocytosis of insulin-containing vesicles [24]. Insulin lowers the blood glucose by promoting glucose uptake and nutrient storage in muscle, fat, and liver [25]. Disturbances in insulin secretion and glucose homeostasis lead to diabetes mellitus, a metabolic disorder characterized by hyperglycemia. In type 1 diabetes (T1DM; “Insulin-Dependent Diabetes Mellitus”), immune-mediated reduction of functional β cell mass results in a diminished or abolished insulin secretion. This results in the inability of the body to maintain normoglycemia. In type 2 diabetes (T2DM) the cause of hyperglycemia is more complex. It ranges from predominantly insulin resistance with relative insulin deficiency to a predominantly insulin secretory defect with insulin resistance. There are several risk factors toward the development of T2DM such as genetic and functional alterations in TRP channels. Furthermore, there is a connection between T2DM and a high-calorie diet or old age [26]. A subset of T2DM patients develops hyperglycemia secondary to hypoinsulinemia instead of peripheral insulin resistance; this is induced by a loss of functional β cell mass [27]. In the next sections, we describe the current knowledge of the function of TRP channels in the regulation of insulin release. TRPC channels are nonselective Ca2 +-permeable cation channels, with the selectivity ratio PCa/PNa varying significantly between the different family members [28]. TRPC channels are widely expressed, and their characterization is complicated by the possible occurrence of heterotetramers. TRPC1 mRNA could be detected in INS-1 cells and rat β cells and at high levels in mouse islets and MIN6 cells, whereas it could not be detected in another mouse insulinoma cell line βTC3 [29,30]. Expression of TRPC1 was found in whole human pancreas [31]. Four transcripts of mouse TRPC1 RNA, representing different splice variants, have been found in mouse islets and the β cell line MIN6 [29]. Polymorphisms in the TRPC1 gene have recently been associated with development of T2DM [32]. However, the influence of TRPC1 on insulin release still needs to be determined. Equally little is known about other TRPC channels in insulin release. Although TRPC4 could be detected in βTC3 and INS-1 cells, rat β cells, mouse islets, and human pancreas [30,31,33,34], analysis of blood glucose homeostasis by glucose tolerance tests did not reveal differences between wild-type (WT) and Trpc4-deficient mice both regarding basal glucose levels under fasting conditions, as well as following intraperitoneal glucose challenge [35]. Because TRPC4 is activated in the phospolipase C pathway, it is possible that the channel is involved in ACh- or glucagon- induced amplification of insulin release. TRPC6 transcripts have been detected, although to a low level, in βTC-3 insulin-secreting cells. Thus, it is clear that more research is needed to clarify the possible function of TRPC channels in the insulin release of the pancreatic β cell. It has been suggested that TRPC channels mediate the unknown depolarizing current that accounts for the Ca2 +-release-activated cation current characterized earlier in βTC3 cells [34]. TRPM2 is a nonselective Ca2 +-permeable cation channel fused C-terminally to an enzymatic ADP-ribose pyrophosphatase domain [36]. The channel is expressed in insulin-secreting cell lines, such as the rat cell lines CRI-G1 and RIN-5 F, and in human and mouse pancreatic islets [14,37–39]. Moreover, the channel coexpresses with insulin, but not with glucagon, indicating expression in β cells [39]. TRPM2 is activated by various stimuli, including adenine dinucleotides (ADPR, cADPR, NAADP, β-NAD), reactive oxygen species (ROS) such as H2O2 and OH−, and intracellular Ca2 + [36,37,40,41]. A current with TRPM2-like properties could be detected in the rat insulinoma cell line CRI-G1, INS-1 cells, and primary mouse β cells. In Trpm2−/− primary β cells, no ADPR-elicited current could be detected, suggesting that TRPM2 is natively expressed and forms a functional channel in β cells [38,42]. TRPM2 could contribute to insulin release induced by heat, glucose, and incretin hormones [39,43]. Indeed, forskolin- (an activator of adenylyl cyclase) and exendin-4- (a GLP-1 receptor agonist) induced insulin release from rat pancreatic islets was significantly reduced in islets after shRNA-mediated knockdown of TRPM2 expression [39]. Furthermore, 2-aminoethoxydiphenyl borate (2-APB), a rapid and reversible inhibitor of TRPM2, inhibits both heat- and exendin-4-evoked insulin release from rat pancreatic islets [44]. These indications are further substantiated in the Trpm2−/− mouse: insulin secretion is induced by glucose, and GLP-1 is impaired in Trpm2-deficient islets, whereas the response to tolbutamide, a KATP channel inhibitor, is unchanged [43]. This results in higher basal glucose levels and an impaired glucose tolerance in Trpm2-deficient mice [43]. The impairment of insulin secretion is caused by reduced increases in [Ca2 +]cyt, indicating that TRPM2 mediates Ca2 + influx on glucose and/or GLP-1 stimulation. However, the situation might be even more complex because glucose-stimulated insulin secretion evoked by diazoxide and high K+ (conditions designed to “clamp” intracellular Ca2 + and inactivate the KATP channel-mediated pathways) was lost in Trpm2-deficient islets. Because the intracellular Ca2 + under these conditions was not altered between WT and Trpm2−/− islets, these data suggest that TRPM2 mediates insulin secretion independent of its role as a Ca2 + entry channel [43]. TRPM2 is important in ß cell apoptosis, a feature linked to the activation of the channel by H2O2 and OH−. These reactive oxygen species that are produced by oxidative stress are thought to play a central role in β cell death and the development of type 1 and type 2 diabetes [45,46]. Indeed, activation of TRPM2 by H2O2 has been shown to mediate Ca2 + influx and β cell death in a rat β cell line RIN-5 F that natively expresses TRPM2 [37,47]. Moreover, INS-1 cells with suppressed TRPM2 expression are 72% less affected by H2O2-induced cell death [42]. The H2O2-induced Ca2 + influx is thought to be mediated by increasing levels of NAD+ or ADP-ribose that bind directly to the Nudix motif in the cytosolic C-terminal of TRPM2 [36,37]. Finally, TRPM2 has been reported to have an additional role as an intracellular Ca2 + release channel in pancreatic β cells [42]. Indeed, internally applied ADPR gives rise to a single Ca2 + transient both in INS-1 and in primary mouse β cells, and this effect was completely abolished in Trpm2−/− primary mouse β cells. Furthermore, TRPM2 colocalizes with lysosome-associated membrane protein-1 (LAMP1), a specific marker for lysosomes. In agreement with this, ADPR-induced intracellular Ca2 + release was abolished in INS-1 cells treated with bafilomycin A, a macrolide antibiotic that empties lysosomal calcium stores without affecting ER stores [48]. These data indicate that ADPR-dependent TRPM2-mediated Ca2 + release occurs predominantly from a lysosomal store. In addition, it was suggested that TRPM2-mediated Ca2 + release contributes to H2O2-induced apoptosis [42]. Indeed, H2O2 induces significant cell death in INS-1 cells in the absence of extracellular Ca2 +, albeit with a reduced severity. This effect was reduced in cells with reduced TRPM2 expression, indicating that not only Ca2 + influx through plasma membrane TRPM2 but also TRPM2-dependent lysosomal Ca2 + release plays a critical role in H2O2-mediated β cell death [42]. The TRPM3 gene encodes for different TRPM3 isoforms due to alternative splicing and exon usage, leading to channels with divergent pore and gating properties [49]. We refer here only to TRPM3α2 (1709 amino acids: the pore lacks 12 aa in comparison to the longest form TRPM3α1 of 1721 aa). Interestingly, the TRPM3 contains a recognition site for miR-204, which may regulate a variety of target genes at the transcriptional level [50,51]. TRPM3 is expressed in a variety of neuronal and nonneuronal tissue [51], including whole pancreas [52,53], INS-1 cells, and mouse pancreatic islets [54,55]. TRPM3 channels are directly activated by the neurosteroid hormone pregnenolone sulphate (PS). Pancreatic β cells and INS-1 cells express PS-sensitive channels that share several pharmacological and biophysical properties of recombinant TRPM3 channels (such as sensitivity to nifedipine and block by monovalent cations [54]). Moreover, PS elicits a large Ca2 + increase in INS-1 cells and pancreatic islets, an action dependent on TRPM3 expression. This PS-induced Ca2 + increase could be blocked by the selective and potent TRPM3 blocker mefenamic acid in INS-1E cells and mouse pancreatic islets [55]. Remarkably, mefenamic acid did not block glucose- or tolbutamide-induced Ca2 + increase, indicating that TRPM3 is not involved in the KATP-dependent Ca2 + signaling of the β cell. PS, however, did increase glucose-induced insulin secretion from pancreatic islets, an effect abolished by mefenamic acid [54,55]. Thus, TRPM3 is an interesting target for the development of insulin secretagogues. Interestingly, PS activation of β cells (via TRPM3 and voltage-gated Ca2 + channels) induces the biosynthesis of a gene regulatory protein, the zinc finger transcription factor Egr-1, and in this way leads to increased biosynthesis of insulin [56]. However, the pharmacological concentrations of PS (50 μM) used to demonstrate enhancement of insulin secretion do not occur in vivo. It is possible that TRPM3 plays a more profound role in conditions where elevated plasma PS levels and changes in glucose homeostasis co-occur, such as pregnancy or 21-hydroxylase-deficiency. TRPM3 channels are also proposed to constitute a regulated Zn2 + entry pathway in pancreatic ß cells [57]. Zinc is important for insulin release as it is packed into cocrystals with insulin in the exocytotic vesicles. The formation of insulin crystals in β cells depends, among others, on the ZnT8 transporter, which contributes to the packaging efficiency of stored insulin [58]. Because Zn2 + ions are coreleased with insulin, pancreatic β cells need to continuously replenish their Zn2 + stores by taking up Zn2 + ions from the extracellular space. Insufficient Zn2 + uptake leads to impaired insulin synthesis and aggravated diabetic symptoms [59]. TRPM3 channels in ß cells have been shown to be highly permeable for Zn2 + and capable of mediating Zn2 + uptake under physiological conditions. The depolarization caused by the activation of TRPM3 channels could lead to the activation of voltage-dependent L-type Ca2 + channels and would in this way also lead to Zn2 + influx through these channels [54,60]. Whether Zn2 + influx regulation via TRPM3 channels is functionally relevant in β cells remains to be elucidated TRPM4 is a Ca2 +-activated nonselective monovalent cation channel that is impermeable to divalent cations. The channel has been proposed to control insulin secretion in a rat insulinoma cell line INS-1, where TRPM4 protein is abundantly expressed [61]. TRPM4 expression and TRPM4-like channel activity could be detected in several β cell lines—INS-1, HIT-T15, RINm5F, β-TC3, and MIN-6—and also in the alpha cell line INR1G9 [12,61]. Inhibition of TRPM4 decreases the magnitude of the Ca2 + signal and insulin release in response to glucose, AVP (arginine-vasopressin, a Gq-coupled receptor agonist in β-cells), and glibenclamide in INS-1 cells [12,61]. These data suggest that depolarizing currents generated by TRPM4 are an important component in the control of intracellular Ca2 + signals necessary for insulin secretion. Furthermore, it is suggested that TRPM4-containing vesicles are translocated to the plasma membrane via Ca2 +-dependent exocytosis, which may represent a regulatory mechanism by which β cells regulate electrical activity [12,61]. However, all these studies have been performed on cell lines. Although TRPM4 protein expression could be found within insulin-producing human β cells and mouse pancreatic islets [12], studies on Trpm4−/− mice revealed no difference in glucose-induced insulin secretion from freshly isolated pancreatic islets [62]. Moreover, these mice did not suffer from an impaired glucose tolerance after an intraperitoneal injection of glucose. These data suggest that TRPM4 is probably not involved in the signal mechanism following glucose stimulation. On the other hand, this does not exclude a possible role for TRPM4 in Gq– or Gs-receptor-coupled signaling pathways, for example during stimulation with glucagon or GLP-1. In addition, TRPM4 is proposed to be involved in glucagon secretion by the pancreatic alpha cell line αTC1-6 [63]: TRPM4 inhibition decreased the magnitude of intracellular Ca2 + signals and glucagon secretion in response to several agonists such as the Gq-protein-coupled receptor agonist AVP and high K+ [63]. TRPM5, like its closest homologue TRPM4, is a Ca2 +-activated nonselective monovalent cation channel that is impermeable to divalent cations [7]. The channel is expressed in β cells from pancreatic islets. A Ca2 +-activated nonselective monovalent cation channel could be measured in β cells and was largely reduced in Trpm5−/− mice, indicating that TRPM5 is an important constituent of the Ca2 +-activated cation current in β cells [64]. The TRPM5-dependent current small, ~ 2pA/pF at –80 mV and 1.5 μM [Ca2 +]cyt [64], suggesting that the channel can only influence the electrical activity of β cells under conditions of high input membrane resistance. Whereas no difference could be detected in Vm or intracellular Ca2 + in nonstimulatory (low glucose, high KATP activity) conditions, TRPM5 seems to influence electrical activity during glucose stimulation (a condition with low KATP activity and consequently a high electrical resistance). Whereas normal WT islets respond to glucose stimulation with three types of oscillations (slow, mixed, or fast), Trpm5−/− islets displayed specifically a lack of fast glucose-induced oscillations in Vm and Ca2 + [64]. TRPM5 contributes to the slow depolarization in the slow interburst interval of the glucose-induced electrical activity, in this way shortening the interburst interval and leading to faster glucose-induced oscillations in Vm and Ca2 +. Why TRPM5 is only functionally relevant in a (fast-oscillating) subpopulation of the islets remains unclear, but it might be that the relative weight of the TRPM5-mediated depolarizing conductance is coupled to the glycolytic rate in the cell. According to the dual oscillator model [23], fast oscillations are characterized by a high glycolytic rate (and a resulting high ATP production). This situation would make TRPM5 activity able to depolarize Vm in the interburst interval, as the hyperpolarizing KATP current is largely inactive at that point. This is in contrast with the situation in slow oscillating islets, where the oscillating glycolytic rate and the resulting high activity of KATP in the interburst interval would make TRPM5 insufficient to depolarize Vm. Fast Ca2 + oscillations are shown to be more efficient than slow oscillations in triggering exocytosis of secretory vesicles and insulin release [65]. In line with this, glucose-induced insulin release was reduced in isolated pancreatic islets from Trpm5−/− mice. Moreover, Trpm5-deficient mice display an impaired glucose tolerance during oral and intraperitoneal glucose tolerance tests [64,66]. This is caused by reduced glucose-induced insulin secretion from the β cells resulting in lower plasma insulin levels. These data suggest that Trpm5- deficient mice display a prediabetic phenotype caused by β cell dysfunction. The relevance of this prediabetic phenotype during conditions of higher insulin demand (such as pregnancy, obesity, aging) remains to be shown. Stimulation of TRPM5 increases the glucose tolerance in mice, by increasing calcium oscillation frequency in islets (Philippaert & Vennekens, unpublished). In leptin-deficient mice, a model for type II diabetes, a vast decrease of TRPM5 expression in the pancreatic islets was observed. Moreover, the glucose- induced calcium activity in islets from leptin-deficient mice resembles those of Trpm5−/− mice [67]. In this regard it is also interesting to note that Trpm5 expression is negatively correlated with blood glucose concentrations in the small intestine from diabetic patients [68]. Moreover, a recent study reports an association of TRPM5 variants with prediabetic phenotypes in subjects at risk for type 2 diabetes [69]. The functional impact of these mutations on the TRPM5 channel activity has not been clarified. However, these data indicate a possible link between TRPM5 and the development of type 2 diabetes mellitus. TRPM8 is a cold sensing TRP channel that is activated by chemical ligands such as menthol and icilin [70,71]. It is highly expressed in prostate tissue; however, its functional role there remains to be elucidated [53]. TRPM8 is functionally expressed in dorsal root ganglia and trigeminal ganglia. In the sensory nervous system, TRPM8 has a prominent role in the sensing of cold temperatures and maintaining core body temperature [72]. There is no expression of TRPM8 in the endocrine pancreas [53]. However, mice lacking TRPM8 have an increased insulin sensitivity, which results from a compensatory mechanism following enhanced insulin clearance in TRPM8−/− mice. This compensatory mechanism includes up-regulation of the insulin-degrading enzyme (IDE) in the liver, which might result from the loss of TRPM8 mediated neuronal signals in hepatic neural innervations. TRPM8 positive sensory afferents innervate the hepatic vein [73]. TRPM8 has recently been indicated in association with pancreatic adenocarcinoma, where it might serve as a potential biomarker [74,75]. TRPV1 is best known for its function in nociceptive neurons, detecting noxious heat and pain. It is activated by heat and capsaicin, the pungent compound in chili peppers [76]. There is functional expression of TRPV1 in the brain and sensory neurons [77]. TRPV1 transcripts have been detected in the pancreas, but it is currently unclear whether there is expression of TRPV1 in the β cells or merely limited to the innervation of the pancreas [78–80]. Treatment of mice with the TRPV1 activator capsaicin increased the postprandial insulin secretion, an effect that was absent in the Trpv1−/− mouse. No direct secretagogue effect of TRPV1 activation on the β cell could be observed. Instead, the insulin-promoting effect of activating TRPV1 can be contributed to increased incretin release from GLP-1 secreting intestinal cells [81]. Antibody reactivity indicates expression of TRPV1 in the afferent neurons innervating the pancreas, but not in the β cells. In nonobese diabetic (NOD) mice, a mutation leading to a hypofunctional TRPV1 channel was identified, which is linked to the onset of T1DM. Congenic NODxB6Idd4 mice carrying a fully functional WT TRPV1 gene do not have a diabetic phenotype. The onset of islet inflammation and diabetes in these mice might be mediated by the TRPV1 + insulin responsive sensory neurons [82]. A similar mutation was found in association with diabetes in a human population [83]. Clearly, TRPV1 could be important to evoke neuronal and hormonal signals to promote β cell activity (see following). However, current knowledge doesn’t support a direct role of TRPV1 in the β cell. Expression of TRPV2 could be detected in MIN6 cells and in mouse primary β cells [84,85]. When MIN6 cells are cultured in a serum-free condition, immunoreactivity of TRPV2 is localized in an intracellular compartment, whereas addition of serum induces translocation of TRPV2 to the plasma membrane [86]. Both insulin and IGF-1 have been shown to be responsible for the translocation and insertion of TRPV2 from an intracellular compartment into the plasma membrane [85,86]. Translocation of TRPV2 was also induced by insulin secretagogues (including glucose) and knockdown of the insulin receptor attenuated insulin-induced translocation of TRPV2 [85]. Furthermore, elevation of Ca2 + entry caused by pretreatment of either MIN-6 cells or cultured mouse β cells with insulin is reduced by inhibition of TRPV2 [85]. Inhibition of TRPV2 also reduces glucose- or KCl-induced insulin secretion [85]. These data indicate that insulin released from the β cells further augments Ca2 + entry by recruiting TRPV2 to the plasma membrane and via this feed-forward mechanism accelerates insulin secretion. Indeed, it has been shown that insulin treatment induces the acceleration of the exocytotic response during the glucose-induced first-phase response by the insertion of TRPV2 into the plasma membrane in a PI3K-dependent manner [84]. Although the autocrine effect of insulin on β cell function has been a matter of debate [87], the β cell specific knockout of the insulin receptor gene shows impaired glucose-induced insulin secretion and reduced β cell mass, showing an important functional role for the insulin receptor in glucose sensing by the pancreatic β cell [88]. In this regard, more knowledge concerning the in vivo role of TRPV2 in β cells would expand the knowledge of insulin signaling in the healthy and the diabetic β cell. TRPV4 is a Ca2 + permeable cation channel [89]. TRPV4 is expressed in MIN6 cells, INS-1E cells, and mouse pancreas [90]. Activation of TRPV4 enhances glucose-stimulated insulin secretion from INS-1E cells [91]. Reduction of Trpv4 expression significantly protected MIN6 cells against hIAPP-induced calcium elevation, ER stress, and apoptosis [90]. hIAPP or amylin, the main component of amyloid, is strongly associated with the progressive loss of pancreatic β cell mass in type 2 diabetes. This may result from disruption of Ca2 + homeostasis. hIAPP forms insoluble aggregates and triggers Ca2 + changes that are associated with the induction of apoptosis via a pathway involving activation of the ER stress response [92]. The cytotoxicity of hIAPP is initiated on the cell surface and requires close contact between the aggregation pores and the β cell plasma membrane [93]. Because TRPV4 might be mechanosensitive, it has been proposed that TRPV4 senses the physical changes in the plasma membrane induced by hIAPP aggregation and in this way enables calcium entry, membrane depolarization, and activation of L-type Ca2 + channels, leading to cell death [90]. TRPA1 is a Ca2 +-permeable nonselective cation channel, which is expressed in trigeminal and dorsal root ganglion neurons. TRPA1 is activated by several reactive electrophilic substances like allyl isothiocyanate (AITC), cinnamaldehyde, allicin, and acrolein, but also by nonreactive compounds like methylsalicylate, menthol, and icilin. TRPA1 is involved in various sensory processes, such as the detection of noxious cold and inflammatory hyperalgesia. TRPA1 is abundantly expressed in a rat pancreatic ß cell line and freshly isolated rat pancreatic β cells, but apparently not in pancreatic alpha cells [94,95]. Application of compounds such as mustard oil and 4-hydroxy-2-nonenal, which activate TRPA1, induces an inward membrane current, depolarization, and a rise of the intracellular Ca2 + level, leading to the release of insulin. This indicates that TRPA1 agonists could function as insulin secretagogues [94,95]. Strikingly, it was also reported that glucose-induced insulin release could be inhibited by the TRPA1 antagonist HC030031 [94]. This might suggest a role for TRPA1 during glucose-induced signaling in the ß cell, though it should be mentioned that data from Trpa1 knockout mice, which are indispensable controls for the selectivity of the antagonist, are lacking at this point. A local feedback loop exists between TRPV1-expressing sensory neurons and insulin-secreting β cells. Insulin released by the β cell will activate insulin receptors on the nerve terminals [96]. This increases TRPV1-mediated membrane currents by enhancing receptor sensitivity and translocation of TRPV1 from cytosol to plasma membrane [96] and by lowering the thermal activation threshold to room temperature [97]. The increase in TRPV1 currents leads to local release of neuropeptides such as substance P and CGRP that will sustain β cell physiology in an optimal range [82]: CGRP reduces insulin release from β cells [98], whereas substance P promotes neurogenic inflammation in the pancreas [99]. This local feedback loop has been proposed to be disturbed in type 1 diabetes mellitus. TRPV1 is highly expressed in primary sensory neurons, where it functions as a polymodal nociceptor [100]. Some controversy exists about expression of TRPV1 in endocrine islet cells. Whereas one study reports the expression of TRPV1 in Sprague-Dawley rat islets and rat β cells lines (RIN and INS-1) and shows capsaicin-induced insulin release from insulinoma cells [78], others failed to show expression in mouse pancreatic islets [82] or in ß cells from Zucker diabetic fatty (ZDF) rat [80]. However, both the exocrine and the endocrine pancreas are innervated by TRPV1-positive neurons [80,101,102]. More and more evidence points to an important role for these neurons in type 1 and type 2 diabetes mellitus [100,103]. Ablation of TRPV1-positive neurons by agonist (capsaicin or RTX) administration in rat neonates improves glucose tolerance in those made diabetic by streptozotocin (STZ) treatment [104]. Nonobese diabetic (NOD) mice that are genetically prone to develop type 1 diabetes carry a hypofunctional TRPV1 mutant that is localized to the Idd4.1 diabetes-risk locus [82]. The hypofunctional NOD mutant causes a decreased secretion of substance P by the pancreatic sensory neurons. Interestingly, direct administration of substance P into the pancreas transiently reverses hyperglycemia in NOD mice and clears islet cell inflammation. The congenic NOD.Idd4.1 mouse strain, which expresses the WT TRPV1, is protected from diabetes [82,105]. However, injecting NOD mice with high doses of capsaicin (and thus eliminating TRPV1-positive neurons) also protects mice from diabetes [82]. Islet infiltration and the proportions and absolute numbers of effector T lymphocytes in pancreatic lymph nodes were reduced in capsaicin treated NOD mice [82]. The different effects of the neuropeptides on β cell function, depending on their concentration, might explain these apparently contradicting results. High concentrations generate a strong trophic signal, promoting β cells survival and function, whereas low concentrations have the opposite result, with deleterious effects on β cell function and viability [106,107]. It has been proposed that a fine balance in the local microenvironment between products such as substance P and islet β cell stress might determine the ensuing inflammatory reactions [82]. Systemic application of capsaicin (or resiniferatoxin [RTX], another TRPV1 agonist) ablates TRPV1-expressing nerve fibers and prevents aging-associated obesity and insulin resistance in rats and mice [108,109]. Furthermore, this treatment prevents the deterioration of glucose homeostasis in Zucker diabetic rats via increased insulin secretion [80]. The channel might also be linked with appetite regulation and obesity (a well-known risk factor for T2DM), as obese monkeys show markedly reduced capsaicin-evoked flare responses in their skin, which may point to down-regulated TRPV1 expression and/or loss of TRPV1-expressing nerve fibers [110]. The anorexic lipid mediator N-oleoylethanolamide is an endogenous TRPV1 ligand that reduces food intake in WT but not in Trpv1−/− mice [111]. As both obesity and T2DM might in part be an inflammatory disorder (as evidenced by increased levels of inflammatory markers [112–114]) and TRPV1 can be activated by inflammatory components [100,115], it has been suggested that TRPV1-expressing nerves are activated by proinflammatory substances in T2DM patients and that the resulting sustained CGRP release and high circulating CGRP levels promote insulin resistance [103]. Interestingly, treatment of ob/ob mice (a mouse model of obesity, insulin resistance, and T2DM) with a TRPV1 antagonist not only enhances insulin secretion but also decreases insulin resistance [116]. Type 2 diabetes mellitus contributes to the development of both macrovascular and microvascular disorders. The major microvascular complications include nephropathy, retinopathy, and neuropathy, whereas the macrovascular complications manifest themselves as accelerated atherosclerosis, clinically resulting in premature ischemic heart disease, increased risk of cerebrovascular disease, and severe peripheral vascular disease [117]. These complications are a frequent cause of morbidity and mortality in patients with diabetes mellitus. Platelet dysfunction, leading to a prothrombotic state, contributes to diabetic microangiopathy and macroangiopathy. Platelets from type 2 diabetic donors show enhanced adhesiveness and ability to aggregate more readily than those from healthy subjects [118]. This platelet hyperaggregability and hyperactivity is associated with abnormal intracellular Ca2 + homeostasis [118,119]. Several studies show that Ca2 + entry induced by the SERCA inhibitor thapsigargin is enhanced in platelets from diabetic donors, suggesting increased store-operated Ca2 + entry (SOCE) [120,121]. TRPC channels are well-known candidates of store-operated channels [122] and are identified in human platelets [123]. Interestingly, expression of TRPC3, Orai1, and STIM1 is enhanced in type 2 diabetes subjects as compared to controls [124]. Furthermore, hyperglycemia has been shown to cause enhanced TRPC6-mediated OAG-induced Ca2 + entry [125]. High glucose increases TRPC6 channel protein expression on the platelet surface, which is mediated by a phosphatidylinositol 3-kinase-dependent pathway [125]. Platelets from patients with type 2 diabetes mellitus showed increased TRPC6 expression as compared to nondiabetic individuals [125]. These data provide an explanation to the enhanced Ca2 + entry induced by physiological agonists in platelets from type 2 diabetes patients. However, reduced expression of TRPC6 in platelets from these patients has also been reported [124]. Moreover, a recent study shows that store-operated divalent cation entry might be attenuated in platelets from type 2 diabetic donors, although the overall Ca2 + entry in these cells stimulated by agonists or thapsigargin is still enhanced [126]. The reduced SOCE might be explained by impairment of the association between the Ca2 + sensor STIM1 and the proteins Orai1, hTRPC1, and hTRPC6 [126]. These data suggest that other noncapacitative Ca2 + entry pathways might be responsible for the enhanced Ca2 + entry observed after platelet stimulation with agonist or the SERCA inhibitor TG. Taken together, the pathogenesis of platelet disorders during type 2 diabetes mellitus remains largely unclear. SOCE is also active in smooth muscle cells and contributes to vasoconstriction [127]. Diabetes is associated with a perturbation of signaling pathways in vascular tissue. This causes vasomotor dysfunction characterized by impaired responsiveness to vasodilators or an exacerbated response to vasoconstrictors, leading to hypertension [128]. Vessels from diabetic patients are more contractile than those from nondiabetic patients [129]. This higher contractility could be partly due to the involvement of Ca2 + entry through the store-operated channels, as well as the regulation of the expression of TRPC1, 4, and 6. In caudal artery strips of type 2 diabetic Goto-Kakizaki (GK) rats, TRPC1 and TRPC6 expression was increased, and TRPC4 expression was found in diabetic, but not in nondiabetic, animals [130]. The situation in humans might be a bit different, as vessels from diabetic patients show up-regulation of TRPC4 but down-regulation of TRPC1 and TRPC6 [129]. Nevertheless, these data suggest that diabetes modulates capacitative Ca2 + entry through the store-operated calcium channel specifically via the regulation of TRPC channels. Diabetic nephropathy is a progressive disorder that causes a decrease in the glomerular filtration rate and is the leading cause of end-stage renal disease. TRPC1 is localized on human chromosome 3q22-24, that is, a region considered to be a hot spot for diabetic nephropathy [131]. TRPC1 gene expression is down-regulated in the kidneys of several animal models of type 1 (STZ-administered rats) and type 2 diabetes (diabetic Zucker diabetic fatty fa/fa (ZDF) and db/db mice) [131,132]. Moreover, TRPC1 protein expression was repressed in kidneys of diabetic patients diagnosed with nodular glomerulosclerosis [131], although no association of TRPC1 polymorphisms with diabetic nephropathy or T2D-associated end-stage renal disease could be found [132]. Thus, TRPC1 dysfunction might be important in the development of diabetic nephropathy. The early stage of diabetic mellitus is characterized by renal hyperfiltration, which promotes the eventual development of diabetic nephropathy. Diabetic hyperfiltration results from a combination of decreased responsiveness of both the renal afferent arterioles and glomerular mesangial cells (MCs) to vasoconstrictors. MCs physiologically regulate glomerular hemodynamics. In diabetes, mesangial contractile function is impaired, and reduced Ca2 + influx is believed to be a major contributing factor to the hypocontractility. Agonist-stimulated Ca2 + entry is significantly inhibited by high glucose in cultured MCs, and this involves a decrease in TRPC6 protein expression. High glucose-induced down-regulation is specific for TRPC6 as TRPC1 and TRPC3 protein expression levels were not affected by high glucose [133]. Diabetic peripheral neuropathy (DPN) is a common chronic complication in early to intermediate stages of diabetes mellitus [134]. It manifests as one or more kinds of stimulus-evoked pain including increased responsiveness to noxious stimuli (hyperalgesia), decreased sensitivity to painful stimuli (hypoalgesia), and hyperresponsiveness to normally innocuous stimuli (allodynia). The mechanisms underlying diabetic neuropathy remain poorly understood. TRPV1 is predominantly expressed in primary sensory nerves [76]. Its activation can result in the release of neuropeptides such as calcitonin gene-related peptide (CGRP) and substance P (SP). Studies with TRPV1-deficient mice demonstrate that TRPV1 is essential for thermal hyperalgesia induced by tissue injury and inflammation [135]. Of interest, treatment with capsaicin, a TRPV1 agonist, has been shown to improve sensory perception in humans with DPN [136], likely because overactivation of TRPV1 with capsaicin leads to Ca2 + overload in and degeneration of TRPV1-positive nerve endings. A similar strategy has been applied recently to the treatment of idiopathic rhinitis in humans [137]. DRG neurons isolated from STZ-induced diabetic rats showed significant increases in capsaicin- and proton-activated currents, implying that painful diabetic neuropathy is associated with enhanced function of TRPV1. This enhanced function involves increased phosphorylation, oligomerization, reallocation of channels to cell surface plasma membrane, and impaired desensitization of TRPV1 [138]. These features contribute to the excitability of sensory nerves that mediate neuropathic pain. In STZ-injected mice, TRPV1 expression varies with the neuropathic phenotype: diabetic neuropathy manifests as an initial phase of thermal hyperalgesia and a late phase of thermal hypoalgesia, and these phenotypes are accompanied by up- and down-regulation of TRPV1, respectively [139]. Moreover, STZ treatment failed to influence thermal nociception in TRPV1-deficient mice [139]. Finally, both TRPV1 expressing sub- and intraepidermal fibers and expression in surviving fibers were shown to be decreased in human diabetic neuropathy skin [140]. Thus, it might be suggested that TRPV1 plays an essential role in DPN. The cardiac muscle is also innervated by TRPV1-positive sensory nerves [141]. Interestingly, protein and mRNA expression of TRPV1 and its main neuropeptides CGRP and SP in the cardiac muscle of STZ-injected type 1 diabetic mice were markedly decreased [142]. It might be suggested that decreased expression of TRPV1 leads to depletion of CGRP and SP in diabetic hearts, which renders diabetic patients prone to myocardial infection, which is a common complication of diabetes mellitus. Central sensitization is also involved in neuropathic pain [143]. The periaqueductal gray (PAG) is a midbrain structure whose role in descending control of pain is quite well established [144] and is an important site for localization and actions of TRPV1 receptors in pain modulation [145]. Activation of glutamatergic projecting neurons in midbrain ventrolateral PAG induces antinociception, and agonists of TRPV1 increase firing of these neurons. The antinociceptive effect of capsaicin injected in the PAG was attenuated in STZ-treated rats, probably caused by a reduction in TRPV1 receptor expression [146]. Thus, down-regulation of TRPV1 expression in this brain area might contribute to diabetic hyperalgesia. Many of the aforementioned results are derived from STZ-induced diabetic animals. It should be noted that precaution must be taken when interpreting these results, as STZ has been shown to have a direct effect on TRPV1 expression and function. Indeed, STZ treatment, irrespective of the glycemic state of the animal, caused increased expression and function of TRPV1 in spinal dorsal horn and dorsal root ganglion, probably via the ROS-mediated pathway [147,148]. The TRPA1 ion channel is expressed mainly in nociceptive primary afferent neurons and is activated by reactive chemicals such as cinnamaldehyde and mustard oil. STZ-induced diabetes increases TRPA1 expression in DRG neurons [149]. Interestingly, the channel is activated by 4-hydroxynonenal (4-HNE) and methylglyoxal, reactive compounds that are generated under hyperglycemic conditions and believed to contribute to the development of diabetic neuropathy [150,151]. This activation is sustained [152], leading to the working hypothesis that sustained activation of the TRPA1 channel by compounds generated in diabetes mellitus contributes to diabetic neuropathy. Indeed, prolonged treatment with the TRPA1 channel antagonist Chembridge-5861528 prevents development of diabetic hypersensitivity and reduces the DM-induced attenuation of the cutaneous axon reflex in STZ-treated rats [152,153]. Interestingly, spinal administration of Chembridge-5861528 produced a marked suppression of diabetic hypersensitivity, whereas cutaneous administration, even at a considerably higher dose, had only a weak antihypersensitivity effect [154]. This finding indicates that the attenuation of diabetic hypersensitivity by a systemically administered TRPA1 channel antagonist is rather due to blockade of spinal than cutaneous TRPA1 channels. It might be that activation of cutaneous TRPA1 channels is important in the induction of diabetic-induced hypersensitivity, whereas spinally located TRPA1 channels play an important role in maintenance of the hypersensitivity [154]. These findings suggest that prolonged treatment with a TRPA1 channel antagonist might prevent development of diabetic hypersensitivity. In this regard, it is interesting to note that paclitaxel, an anticancer drug that is known to potentiate cold hyperalgesia in diabetic patients, has been shown to activate TRPA1 via the production of ROS [149]. Whereas in T1DM insulin administration is the most important treatment, the most obvious treatment for T2DM is a change in lifestyle, accomplished by adaptation of exercise and diet. However, in many cases these measures fail to control the elevated blood glucose, and additional treatments are needed [155]. Historically, sulfonylureas were often used, but these drugs come with many unwanted effects, including the risk for hypoglycemia, weight gain, and exhaustion of the pancreas. Currently, the first-line drug of choice is metformin, an oral antidiabetic drug affecting peripheral insulin resistance. However, as beta-cell dysfunction is also associated with type 2 diabetes, antidiabetic drugs should also improve beta-cell function. TRP channels might be interesting drug targets in this regard. TRPM3 activators have been shown to enhance glucose- and GLP-1-dependent insulin release. TRPV1 might also be an interesting drug target as compounds modulating channel activity influence insulin resistance but also insulin release, GLP-1 secretion, and diabetic nephropathy in mice. Indeed, considering that multiple TRP channels are also involved in diabetes-associated complications (as outlined earlier), pharmacological targeting of these channels could affect both insulin secretion of the ß cell and diabetes-associated complications in peripheral tissue. Considering the side effects of sulfonylureas, novel insulin secretagogues are obviously wanted. An essential requirement for a new type of these drugs would be the glucose dependency of its action to avoid the risk of hypoglycemia. Incretin mimetics (GLP-1 receptor agonists that bind specific receptors and mimic the action of natural GLP-1) and incretin enhancers (inhibitors of the enzyme that degrades the incretin hormones and thus prolong their activity) are potential candidates. However, GLP-1R agonists require subcutaneous administration, and both incretin mimetics and enhancers are associated with unwanted effects, such as nausea and diarrhea for incretin mimetics and higher occurrence of infections for incretin enhancers [156], Furthermore, pharmacological activation of TRPM5 would enhance insulin release only under conditions of elevated glucose levels with no associated risk to hypoglycemia. As TRPM5 is also implicated in transduction of sweet taste, activators of TRPM5 might be expected to increase sweet sensation and in this way reduce intake of sugar. Type 2 diabetes has a tremendous impact on public health worldwide. It is generally acknowledged that the disease results from a combination of peripheral insulin resistance and genetically determined susceptibility to β cell dysfunction. There is good evidence that TRP channels can regulate insulin release and β cell function. Specifically, through their ability to regulate intracellular Ca2 + levels and membrane depolarization, TRP channels might be interesting targets for the development of insulin secretagogues. TRP channels might be even more important drug targets in pathology secondary to diabetes, such as diabetic neuropathy, vasculopathy, and nephropathy. Although much of the available evidence is generated in cell lines, data are increasingly being confirmed in knockout mice. However, a functional role for any TRP channel in human islets has not been shown to date. This requires selective and potent pharmacology, which is only available for a few TRP channels. Clearly, this is one of the main hurdles that need to be taken to fully explore the potential of TRP channels in different aspects of diabetes mellitus.
Transient Receptor Potential (TRP) Cation Channels in Diabetes
* Corresponding author: Koenraad.Philippaert@med.kuleuven.be
Abstract
Acknowledgments
TRP’s in Pancreatic ß Cells
TRPCs
TRPM2
TRPM3
TRPM4
TRPM5
TRPM8
TRPV1
TRPV2
TRPV4
TRPA1
TRPV1-Expressing Sensory Neurons in β Cell Function and Diabetes Mellitus
TRPV1 in Type 1 Diabetes Mellitus
TRPV1 in Type 2 Diabetes Mellitus
TRP Channels in Diabetes-Associated Complications
TRPCs in Diabetic Vasculopathy
TRPCs in Diabetic Nephropathy
TRPV1 and TRPA1 in Diabetic Neuropathy
TRPV1
TRPA1
TRP Channels as Drug Target in T1DM and T2DM
Conclusions
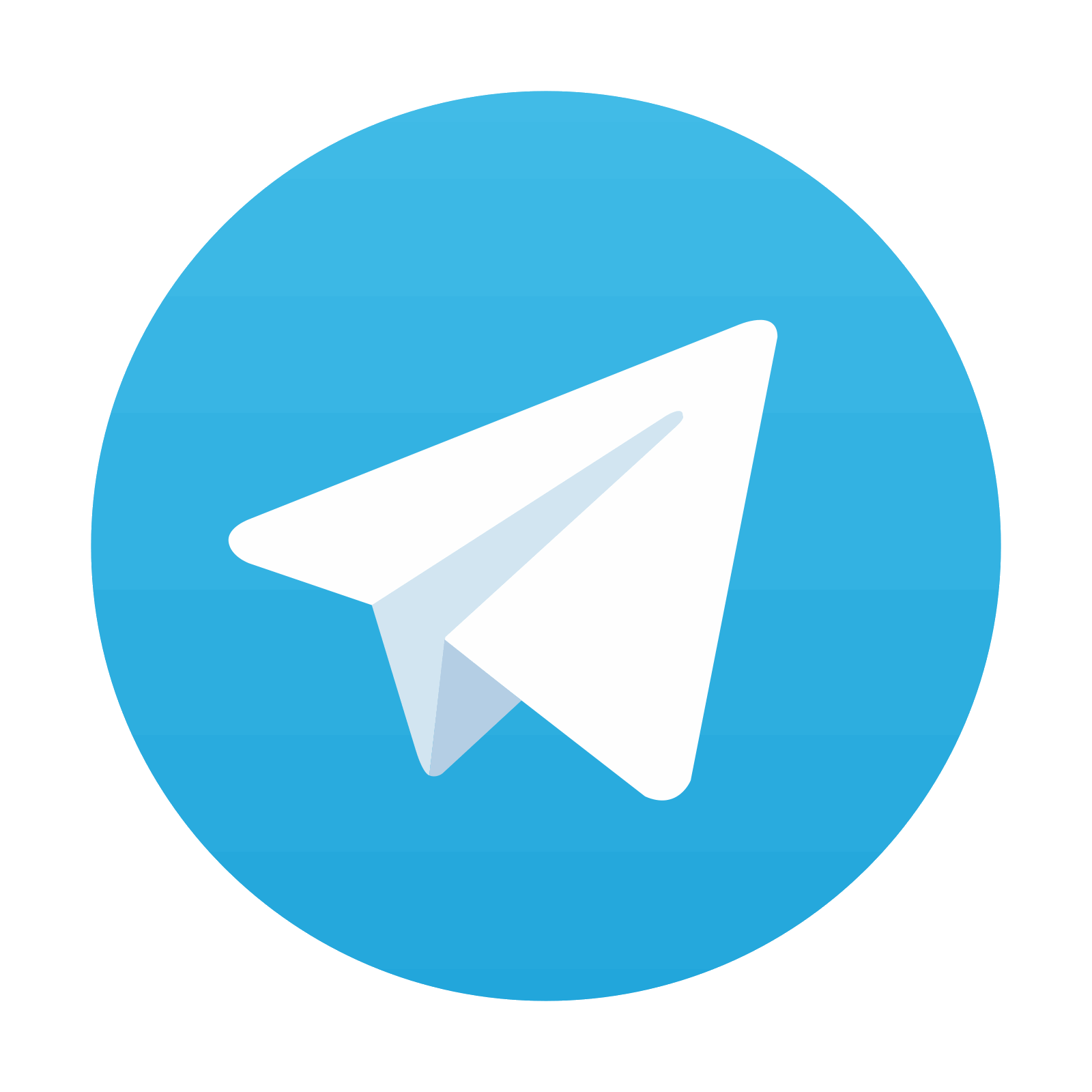
Stay updated, free articles. Join our Telegram channel
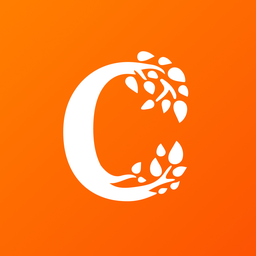
Full access? Get Clinical Tree
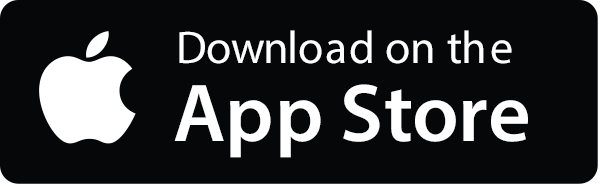
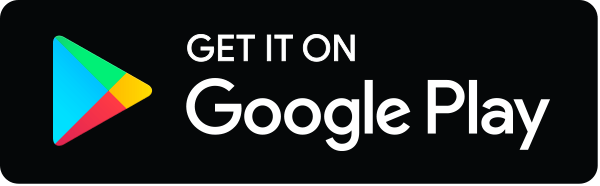