and Miguel A. R. B. Castanho2
(1)
Instituto de Bioquímica Médica Leopoldo de Meis, Federal University of Rio de Janeiro, Rio de Janeiro, Rio de Janeiro, Brazil
(2)
Institute of Biochemistry and Institute of Molecular Medicine, School of Medicine, University of Lisbon, Lisbon, Portugal
In a cell, the fluxes of matter and energy are highly controlled so that cells can maintain their organization and multiply when needed. We have discussed in the previous chapter that consecutive reactions can be driven through coupling favorable reactions to unfavorable reactions, many of those benefiting from the ΔG o of ATP hydrolysis. Synthesizing ATP involves using energy associated to the chemical processing of nutrients or molecules stored for this purpose (catabolic metabolism ). When in excess, the nutrients tend to engage a series of reactions whose end products are the storage molecules (anabolic metabolism) for later use. This shift implies a complex network of metabolisms that must be inhibited or activated. Inhibition and activation occur selectively at specific reactions which in turn occur in specific locations and precise timings inside the cells. In addition, the shift requires that different cells in the same tissue or in different tissues operate coordinately. Liver, adipose tissue, muscle , and brain, for instance, need to be coordinated so that when the brain and muscle require specific nutrients to operate, this process does not conflict with processes in other organs and no failure of the body function as a whole occurs.
Only specific selected sets of reactions take place at a given time in each organelle of a cell. Chemical entities (hormones) circulate in the body and are captured by cell receptors that trigger short chemical reaction sequences, generically named “signal transduction pathways” (see Box 5.1 and Fig. 5.1) upon binding. In the end, these short sequences of chemical reactions modify enzymes stimulating or inhibiting the catalysis of metabolic reactions (Fig. 5.1). The same hormone may be sensed by different cells in different organs. The metabolic events triggered in different organs are not necessarily the same but are coordinated. For instance, during prolonged aerobic exercise, muscles are consumers of fatty acid s , which must be mobilized from the adipose tissues; in this situation both the muscle and the adipose tissue are not activating the same metabolic pathway s , but they are certainly coordinated. A drop in glycemia (glucose concentration in blood) leads to the release of glucagon , a peptide hormone synthesized in the pancreas that binds to receptors in hepatocytes (liver cells) and triggers events that activate reactions leading to the production of glucose (see Chap. 9). Glucose is then released in the blood through the optimized mesh of capillaries throughout the whole liver. This matter will be revisited later in Box 8.1.
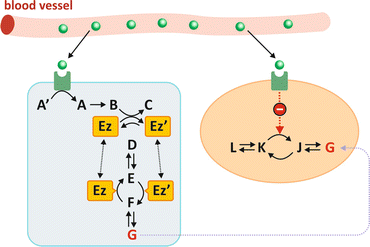
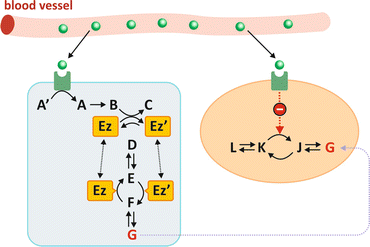
Fig. 5.1
When the hormone (green sphere) binds to its receptor, which may be located on the membrane or may be intracellular, this translates into a short series of chemical reactions (exemplified as compounds A′ to C) named “signal transduction.” One of the consequences may be that one enzyme is modified (Ez to Ez′ in the picture). This transformation may be phosphorylation , for instance (Ez′ being in this case Ez with a covalently bound phosphate group). In this hypothetical situation in which Ez and Ez′ catalyze opposing reactions irreversibly, the practical effect of the hormone is to dictate the sense of metabolic reactions. In the case depicted in the figure, the presence of the hormone in blood generates metabolite G into the cell on the left. The same hormone may be acting at the same time in different cells (colored light blue and salmon) in different organs triggering different metabolic pathway s . The hormone may stimulate G-consuming pathways in cells from other organs (e.g., leading to inhibition of the enzyme that converts K in J)
Box 5.1: Biosignaling, the Communication Among Cells and Inside Cells
In a complex organism, such as the human body, having specialized systems, with specialized organs, specialized tissues, and specialized cells, coordination requires fine-tuning and reliability. The homeostasis of the human body requires that the action of different organs is not conflicting. Imagine a situation such as prolonged starvation. The liver synthesizes glucose and releases glucose in blood to keep the glycemia within safe levels for the brain to operate. What would happen if other organs such as striated muscle were subtracting glucose from the blood to synthesize glycogen , for instance? This conflict between liver action and striated muscle action would be fatal or, at least, result in a paramount waste of energy and matter. There are mechanisms that prevent conflicts of this kind. These mechanisms coordinate the action of cells that may be in contact with each other in the same tissue or in very remote locations relative to each other, such as different organs. There are molecules that serve as “signals” that are released and trigger synchronized and compatible events in different cells. This is known as cellular communication or biosignaling.
Naturally, biosignaling, such as any communication process, requires that there is a source for the signal (e.g., hormone ), the means for the signal to disseminate, and a receptor, or receptors. The signal is a molecule that is synthesized, so the steps of biosignaling are (1) synthesis of the molecule that will serve as signal; (2) release of the signal molecule; (3) transport/dissemination to the target cells, i.e., cells with the receptors that bind and are responsive to the signal molecule; (4) interaction with the receptor, usually a protein , in the target cell; (5) triggering intracellular chemical or physical events that result directly from signal–receptor interaction (“signal transduction”); and (6) generation of other events, frequently a series of events (“cascade” or “signaling pathway”), which constitutes the final response of the cell to the signal.
Hormones , neurotransmitters , prostaglandins, growth factors, and cytokines, such as interleukins and interferons, serve as biochemical communication signals. These signals may act at short range or long range (see figure). Short-range communication involves diffusion of signals in the extracellular medium in the immediate vicinity of the cell that secretes the signal molecule. This is named paracrine signaling . Synaptic signaling is an example of paracrine signaling: neuronal termini release neurotransmitters that bind to receptors in the postsynaptic cells. Long-range signaling is known as endocrine signaling. In this case, specialized cells in specialized organs synthesize hormones, which are usually released into the bloodstream and distributed throughout the body. Hydrophilic hormones are soluble in blood and are easily distributed; hydrophobic hormones might need transporters and/or have severe limitations in the concentrations they can achieve in blood. Steroid hormones, for instance, are hydrophobic hormones derived from cholesterol , and they need to be associated to carrier proteins to be distributed in the body through the blood.
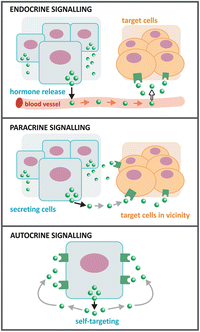
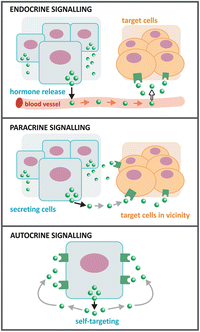
A third form of signaling exists in addition to endocrine and paracrine: autocrine. However, this signaling is mainly found in pathological conditions such as cancers. Tumor cells release growth factors that bind to receptors in the surface of the same cell that releases them, stimulating cell growth and cell division. These events become unbalanced and the tumors grow uncontrolled.
Most molecular signals have very high selectivity and affinity for their receptors. High selectivity means that the receptor is responsive only for a very precise structure of the ligand , i.e., the molecule that serves as signal . High affinity means that the binding equilibrium of the ligand signal to the receptor is very extensively shifted toward the bound ligand–receptor complex, which in practice means that very low concentrations of the ligand generate an integrated high response from the receptor. Nanomolar (10−9 M) concentration of hormones usually suffices to trigger physiological responses because the high affinity for the receptor compensates the low concentration of the ligand.
Depending on whether the hormones may translocate through the plasma membrane or not, the receptors may be located on the surface of cells, or inside the cells, in the cytoplasm or nucleus. Adrenalin, insulin , and glucagon , for instance, have surface receptors; in contrast, testosterone and progesterone, for instance, bind to receptors intracellularly.
Upon binding of the ligand to receptor, the signal transduction process may be of three main different types depending on the functionality of the receptor:
1.
When the receptor is an ionic channel , the ligand may activate or inhibit the flux of ions through the channel. This is the case of the receptor of acetylcholine in the neuromuscular junction.
2.
When the receptor is coupled to G protein (guanosine nucleotide -binding proteins ), binding of the ligand causes conformational changes in the receptor that indirectly activate the G protein , which detaches it from the receptor and binds to adenylate cyclase (also known as adenylyl cyclase) or a phospholipase or another enzyme that catalyzes the formation of the molecules so-called second messengers. Second messengers then initiate series of reactions that will interfere with metabolic processes. Cyclic AMP , cyclic GMP, inositol triphosphate, and diacylglycerol are examples of second messengers, and the receptors of adrenaline and serotonin are examples of G protein-coupled receptors.
3.
When the receptor has catalytic tyrosine kinase activity, binding of the ligand causes conformational changes that make the enzyme active and thus able to phosphorylate proteins in Tyr residues using ATP as a source of phosphate. The insulin receptor is of this kind.
The intracellular signaling pathways that follow the binding of the signal molecule to the receptor are diverse. The main signaling pathways associated to the regulation of metabolism are addressed individually in the main text.
Glycemia is a key factor in energetic metabolism as the brain uses exclusively glucose as a source of ATP production (the only known exception occurs in long-term starvation; see Sect. 9.3.4), and there is no mechanism to keep a higher concentration of glucose in the central nervous system than in blood because glucose transporters across the blood–brain barrier do not operate against a concentration gradient. Therefore, the coordination of metabolisms occurring in different organs simultaneously is such that glucose concentration in the blood is kept higher than a specific threshold. Values under this threshold produce loss of conscience, coma and death may occur (Fig. 5.2). Given the importance of glycemia control and the investment represented by regulatory pathways it is not surprising that biochemists tend to overemphasize the importance of glucose as a nutrient compared to amino acid s and lipids . Yet, lipids and proteins are the most important energetic reserves in the human body (see Table 9.1). Energetic metabolisms are often but erroneously associated to carbohydrate metabolism only. In reality, fatty acid , amino acids, and glucose metabolisms are interconnected. But despite the connections, the metabolites pertaining to each pathway are not necessarily interconvertible. Lipids are typically storage molecules so they cannot be converted to glucose or proteins; it can only be converted in metabolites that in principle will follow reaction routes leading to ATP synthesis (Fig. 5.2).
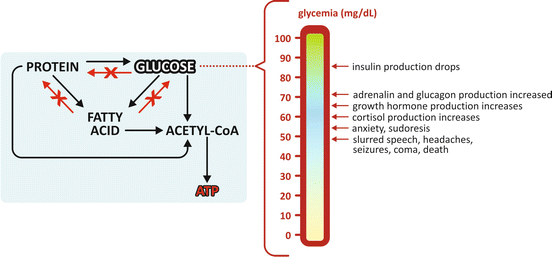
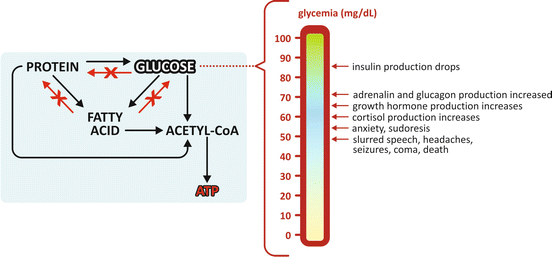
Fig. 5.2
Allowed and forbidden conversion routes in human metabolism . Glucose and fatty acid s cannot be converted to proteins . Fatty acids cannot be converted to proteins or glucose . This implies that all excess nutrients from food intake end in lipids , stored in adipose tissue. Glycemia (the concentration of glucose in blood) needs to be kept above a certain threshold, even in the absence of food intake. In this case, synthesis of glucose is possible using amino acid s from muscle protein degradation
In summary, metabolisms need regulation both inside the cells and in different tissues, frequently in different organs. Therefore, different levels of regulation exist. They are associated to mechanisms with different efficacies, time scales, and areas of impact.
5.1 Levels of Regulation: Impact and Time Scale
Think about your simplest daily routines, like eating, moving, and sleeping. They seem extremely banal and simple, almost unnoticeable in our lives, but they are demanding challenges from the metabolic point of view. They involve relatively fast alternation between states of nutrient abundance (meals) and nutrient absence (fasting), rest (e.g., sleeping), moderate exercise (e.g., walking), exercise bursts (e.g., short run to catch a bus) or intense enduring exercise (e.g., athletic running or swimming), and all possible combinations of feeding state with exercise. At the same time, there are processes that constantly consume energy such as brain activity (any basal activity, not only mental work), heartbeat, or keeping body temperature. Metabolic adaptation to fluctuating conditions on top of basal permanent activities requires mechanisms of metabolic regulation that are (1) fast, (2) efficient, and (3) robust. In fact these factors are not independent: robustness comes from redundancy of mechanisms—having more than one mechanism to assure the same effect decreases the chances of failure; redundancy is a cooperative combination of similar mechanisms—a fast one, albeit not so efficient, and a very efficient one, albeit not very fast. Usually fast regulation mechanisms consists in controlling the availability of substrates and/or the activity of enzymes , while very efficient regulation mechanisms consist in controlling the presence of enzymes, i.e., their genetic expression, which is naturally a slower process. Genetic expression is usually dependent on hormones, which guarantee coordination among the metabolism in different organs, all under the influence of the same circulating hormones. This interplay between mechanisms is illustrated in Fig. 5.3.
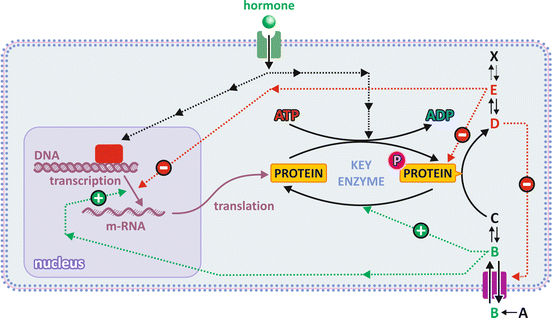
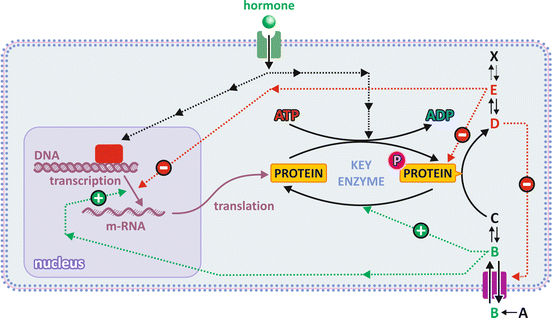
Fig. 5.3
The different levels of regulation are (1) substrate availability through control of transport across membranes (metabolite B); (2) enzyme activation by upstream metabolites (B) in the metabolic pathway and/or enzyme inhibition by the product of downstream metabolites (E) in the metabolic pathway; (3) activation or inactivation of enzymes by covalent attachment of a phosphate group (P), which is usually the end effect of a cascade of events caused by the binding of a hormone to its receptor (signal transduction); and (4) regulatory enzymes’ expression/translation controlled by hormones through signal transduction inside cells. Hormone-dependent mechanisms are slower; the direct effect of metabolites on enzymes (activation or inhibition) is faster because enzymes coexist with regulatory metabolites (named effectors) in the same cell compartment
5.2 Inhibition and Activation of Enzymes by Ligands
As mentioned in the previous section, controlling the activity of enzymes (i.e., changing their kinetic characteristics such as K M or V max) is a fast way to influence the rate and course of metabolic pathway s . Physical factors such as temperature and pH have a direct effect on the structure of proteins because they alter the intramolecular forces that stabilize protein folding. Therefore, they affect the activity of enzymes. Enzymes have optimal temperature and pH ranges to operate (Fig. 5.4). Below and above the optimal ranges, the reaction velocity decreases. Human enzymes are adapted to body temperature and have optimal activity around 37 °C. They are also adapted to the pH of their micro-environments. Pepsin and other stomach enzymes, for instance, have optimal activity at acidic pH.
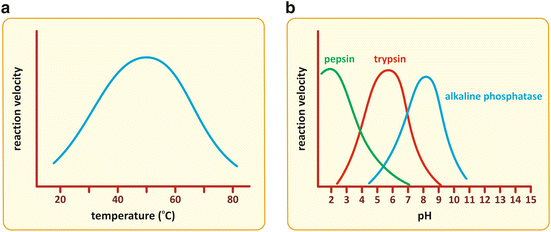
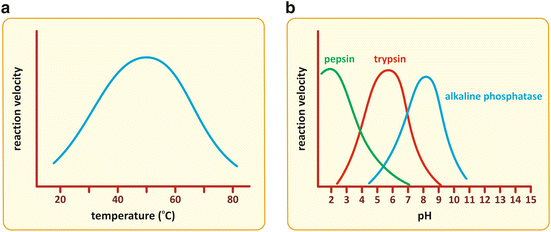
Fig. 5.4
Enzymes suffer structural alteration in their folding when physical factors such as temperature (a) or pH (b) change, which in turn cause alterations in the kinetics of catalysis. The reaction velocity is maximal in a limited interval of temperature or pH, decreasing for higher or lower values. Enzymes are adapted to the local pH of the different micro-environments of the human body from very acidic (such as pepsin) to alkaline (such as alkaline phosphatase ), as illustrated in panel (b)
There are microorganisms living in extreme environments such as hot springs, at low pH and high temperatures. They are called extremophiles for this reason. Nevertheless, even in these cases, there is adaptation of the enzymes to the local pH and temperature. These molecules are very appealing to the biotechnological industry because they can be used in industrial processes that combine extreme pHs with high temperature; however, they are not in the realm of human biochemistry and will not be further discussed here.
In principle enzymatic activity could be modulated by changing pH or temperature, but this is not an option as these factors do not affect specifically a single enzyme in a specific metabolic pathway . The inhibition or activation of an enzyme has to be very selective. Shutting down a metabolic pathway demands inhibition and/or activation of very specific enzymes (recall Sect. 4.2.1), which is not compatible with the manipulation of pH or temperature. Instead, having small molecules that bind specifically to unique binding sites of enzymes, affect their conformation, and influence their kinetics is a much better way to specifically modulate the activities of selected enzymes. These small molecules are called ligands , and they can slow (inhibitors ) or accelerate (activators) the catalytic process.
In the case of an enzyme obeying Michaelis–Menten kinetics, ligands may in principle affect V max, K M, or both. The molecular mechanisms behind the influence of ligands on V max or K M may be very diverse, even if the end result is the same. Two different inhibitors may impact on V max, for instance, binding to different sites of the same enzyme and producing different effects on enzyme structure. In other words, the alterations ligands cause on kinetic parameters tell nothing about how ligands and enzymes interact. To relate kinetics with mechanism of interaction, one has to resort to models, i.e., hypothetical arrangements and postulated events, and deduce on the final in kinetics.
This means that the examples in Box 4.4 can be extended to the case in which an additional molecule, the inhibitor or the activator, interacts with the enzyme Ez, in addition to S (the substrate) and P (the product). Let’s work on a simple example. Postulating that there is a ligand that binds to Ez and prevents S from binding to Ez (in this case the ligand acts as an inhibitor and will be represented by I), the reaction scheme is composed of


The action of I is to prevent part of Ez to take part in the reaction. The effective concentration of Ez available to interact with S is decreased, and naturally, K M appears to be increased. Having less extensive binding of Ez to S appears to be a decreased affinity of Ez to S. In reality the intrinsic binding of Ez to S is not affected in its nature; it is the “sequestration” of Ez by I that causes the alteration in K M. For very high S concentration, S is so abundant relative to I that, in practice, the influence of I on binding of Ez to S is not relevant and so V max remains constant. V max is k 2.[Ez]0 (Box 4.4) and I do not change k 2 or [Ez]0. The reaction velocity vs. [S] plot shows a decreased slope at small [S] but the same asymptotic value (V max) in the presence of I when compared to the plot in the absence of I (Fig. 5.5).
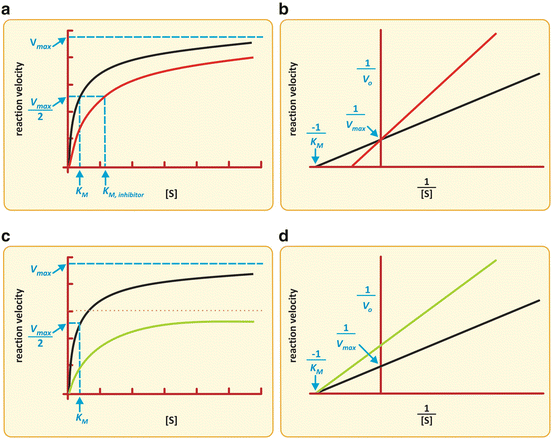

(5.1)

(5.2)
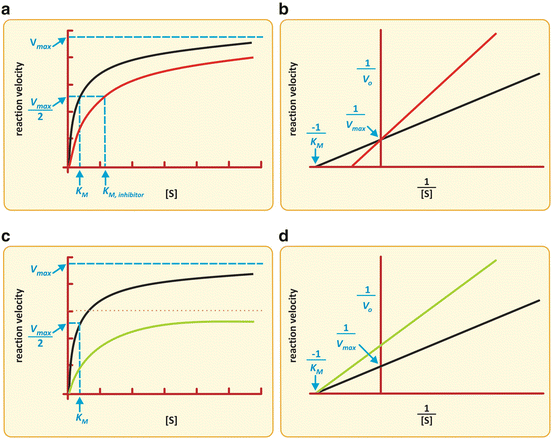
Fig. 5.5
Two possible effects of an inhibitor on the Michaelis–Menten kinetics of an enzyme . (a, b) K M is increased with no alteration in V max. (c, d) V max is decreased with no alteration of K M. Simultaneous alterations on V max and K m are also possible (not shown), which are the most frequent situations in practice
If I binds to Ez irreversibly
, part of enzyme population is permanently blocked by I, so the effective concentration of Ez free to interact with S is decreased even at high concentrations of S. In this case, V max is decreased (recall again V max = k 2·[Ez]0—Box 4.4).
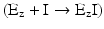
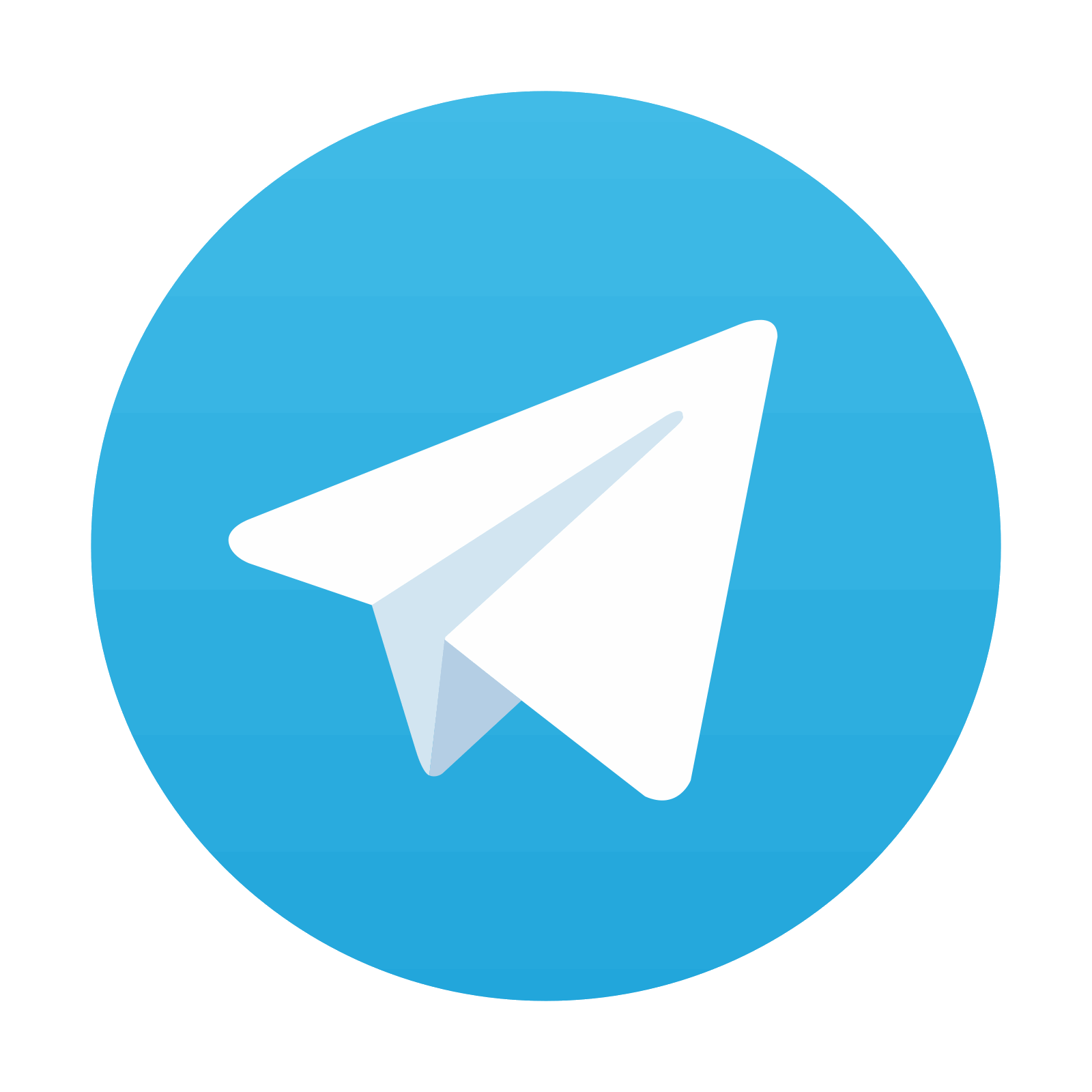
Stay updated, free articles. Join our Telegram channel
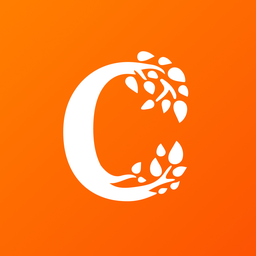
Full access? Get Clinical Tree
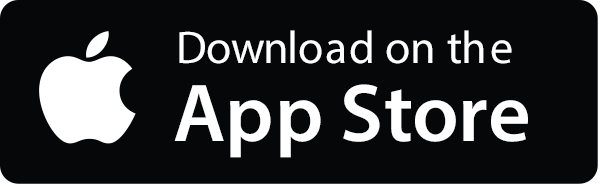
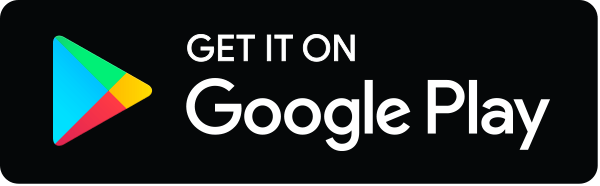