Fig. 21.1
CD4+ T-cell differentiation, cytokine production and their implications in EAE and MS. Naïve CD4+ T cells exit the thymus and upon interactions with antigen-presenting cells they differentiate into different CD4+ T helper lineages depending on the cytokine milieu present. These lineages include Th1, Th2, Th17 and Tregs, which are naturally derived from the thymus or induced in the periphery. Each lineage is under the control of different transcription factors and defined by the particular cytokines they produce. Th1 and Th17 cells are known to be pro-inflammatory T cells that initiate and potentiate EAE and MS, while Th2 and Treg cells are anti-inflammatory and help suppress and reverse disease severity
A new CD4+ T-cell lineage involving IL-23 and IL-17 has now been implicated as playing a prominent role in the pathogenesis of EAE [47]. This new subset, termed T helper 17 (Th17) cells, secrete IL-17, IL-21 and IL-22 and their differentiation is under the control of transforming growth factor-beta (TGF-β), IL-6 and IL-23 [47–49] (Fig. 21.1). Th17 cells are able to potentiate autoimmune responses in EAE [50] and induce EAE when adoptively transferred into mice [48]. In line with these results, IL-17-deficient mice develop less severe EAE compared to wild-type littermates [51] and neutralizing antibodies directed against IL-17 ameliorates EAE [52]. IL-17 has also been implicated in MS, with increased numbers of IL-17 transcripts detected in chronic MS lesions compared with acute lesions or control tissue from patients [53]. Interestingly, similar to Th17 cells, TGF-β is also required for the development of T regulatory (Treg) cells [54] (Fig. 21.1), suggesting some developmental plasticity between Th17/Treg lineages. The induction of a transcription factor from the forkhead box family, FoxP3, by TGF-β is critical for Treg development [55], but the presence of IL-6 can inhibit FoxP3 upregulation and skew T-cell differentiation toward a Th17 phenotype [49, 54]. Therefore, it was proposed that IL-6 production may be important in controlling the extent of inflammation in EAE, via the Th17/Treg balance [56]. Tregs are generally classified as being CD4+ CD25+ T cells, however in recent years it has become apparent that FoxP3 is necessary for the development and function of naturally occurring Treg cells [57–59]. It is believed that during T-cell development in the thymus, naturally occurring Tregs positive for FoxP3 are delivered into the periphery, where they make up 5–10 % of CD4+ T cells [60]. To add to the complexity of these peripheral tolerance mechanisms, naturally occurring FoxP3+ Tregs are not the only regulatory T cells present. Many other regulatory T cells have been described including TGF-β producing T helper 3 (Th3) cells, IL-10 producing type 1 T regulatory (Tr1) cells, CD8+ CD28− T cells, γδ T cells and NKT cells [61–63].
4 Neurodegeneration in MS
It is now recognized that in addition to demyelination, progression of MS leads to considerable damage to neurons, which results in brain atrophy and cumulative disability. Furthermore, axonal degeneration has been shown to directly correlate with both permanent disability and brain atrophy in advanced MS [64]. However, it is well documented that in RR-MS, demyelination in early MS is not permanent and remyelination by oligodendrocytes occurs [64]. This suggests that the brain does possess endogenous repair mechanisms, however, over time these mechanisms either fail or are no longer sufficient to repair the ongoing damage.
There are many factors that contribute to the failure of the CNS to repair itself, these include the physical barrier created by the astroglial scar and the release of growth inhibitory factors that inhibit the migration of progenitor cells and not only prevent myelination, but also axonal regeneration. These inhibitory factors can be produced by a number of cells including oligodendrocyte precursor cells (OPCs), microglia and meningeal fibroblasts [65]. Furthermore, myelin debris found in lesioned areas also contributes to the inhibition of axonal regeneration [66]. Five myelin proteins have so far been characterized with inhibitory activities, these include: Nogo A, oligodendrocyte myelin glycoprotein (OMgp), myelin-associated glycoprotein (MAG), semaphorin 4D, and ephrin B3 [67]. Signaling by these proteins results in growth cone collapse, which usually drives the extension of the growing axon toward its target.
It is well known that the brain has limited plasticity, however, endogenous OPCs have been identified in the adult brain [68]. Moreover, OPCs have been identified in chronic MS lesions and they appeared to interact with dystrophic axons but were unable to remyelinate these damaged axons [69]. The mechanisms behind this failure of endogenous oligodendrocyte repair are not fully understood, but are believed to be due to the presence of myelin inhibitory proteins as well as the presence of the astroglial scar, the insufficient recruitment of OPCs and deficiencies in their ability to differentiate into myelinating oligodendrocytes. Multipotent progenitor cells, including OPCs and neural progenitor cells (NPCs), reside in specific areas of the CNS including the subventricular zone. The migration of OPCs is regulated by a number of chemoattractants and chemorepellants. Cues from growth factors such as platelet-derived growth factor-a (PDGF-a) recruit OPCs to areas of damage in the CNS where further stimuli are required for proliferation, differentiation, and myelination. The breakdown of the BBB in MS results in exposure of progenitor cells in the SVZ to additional stimulatory molecules that direct their migration, such as nerve growth factor (NGF) [70]. NGF, while playing an important role in differentiation and survival of neurons, also stimulates oligodendrocyte differentiation. Treatment paradigms that can enhance this already endogenous reparative process will therefore be important in improving the clinical outcome for MS patients.
5 Current MS Therapies
Significant efforts are being invested into developing novel therapies for MS and understanding the mechanisms by which such therapies have their effects, as there is at present no cure for this disease. Nonetheless, most of the current drugs that are used in the clinic act in an immunomodulatory or immunosuppressive manner [5, 71, 72], with the primary aim of disabling the immune system. This can be achieved in a number of ways, including targeting whole populations of immune cells involved in the disease pathology (i.e. activated T cells), blocking migration of peripheral lymphocytes into the CNS or “resetting” the immune system by deleting and replacing the existing immune cell repertoire [72]. Currently available treatments used for RR-MS in Australia include interferon-beta (IFN-β) preparations (Avonex, Betaferon and Rebif), glatiramer acetate (Copaxone), natalizumab (Tysabri), Fingolimod (Gilenya) and Teriflunomide (Aubagio) with dimethyl fumarate (Tecfidera) currently under review by the Therapeutic Goods Administration. Although IFN-β preparations and glatiramer acetate treatments have proven to be safe with long-term application and lead to a reduced number of relapses [73], nearly 70 % of RR-MS patients do not respond and little effectiveness has been shown in patients with progressive forms of MS [72, 74, 75]. Natalizumab is a more potent therapy but this is tempered by a risk of progressive multifocal leukoencephalopathy (PML) [72]. Other humanized monoclonal antibodies that can specifically block molecules relevant to the pathogenesis of MS are completing clinical trials: these include Daclizumab (Zenapax) [76–78], Rituximab (Rituxan) [79, 80], and Alemtuzumab (Campath) [81–83]. Fingoloimod and Teriflunomide specifically target lymphocytes, known to play a role in disease pathogenesis, and have also shown moderate efficacy [84–88]. However, a fundamental issue with these newer treatments is that while they deplete or functionally inhibit pathogenic immune cells, they also have the same effect on the normal immune response. This consequently leaves the patient immunocompromised, as has been demonstrated with deaths from disseminated herpes and varicella. For this reason there is a clear need for improved therapies that are aimed at providing more specific “targeted” treatments, as well as incorporating neuroprotective strategies to prevent the progression of disease to chronic disability. The remainder of this review will be exploring the potential of a new treatment strategy that may help in overcoming some of the pitfalls we face with current therapeutic approaches for MS, stem cell therapy holds the promise of not only offering immune suppression, but also potential neuroprotection and neuroregeneration.
6 Potential of Stem Cell Therapy for MS
Stem cells are characterized by their multipotentiality and their capacity for self-renewal, which is purported to be essential for their role in the development, maintenance, and repair within specific tissue niches [89]. Given that stem cells are also highly immunosuppressive [90] they are, in principle, ideal for targeting the inflammatory process in MS, in addition to potentially promoting and/or enhancing spontaneous remyelination [13]. Transplantation of human and murine-derived mesenchymal stem cells (MSCs), as well as neural precursor cells (NPCs), has been shown to ameliorate clinical signs of EAE as well as to reduce CNS pathology [13, 71, 90–95]. It has also been shown that when MSCs are injected intraventricularly into EAE mice they traffic to areas of CNS inflammation [94]. A large international multicenter clinical trial is currently underway to assess the safety and efficacy of MSC in treating active RR-MS [96].
However, there are limitations in the therapeutic usage of MSCs. These include the requirement of invasive procedures to acquire these cells by bone marrow biopsy, as well as the low number of adult stem cells that can be isolated from adult tissues, necessitating extensive culture expansion. This restricts their use for autologous transplantation in adults. A novel type of stem cell that is gaining interest among the stem cell research community as a potential alternative are human amnion epithelial cells (hAECs). The advantage of these cells is they are easily obtained in large numbers from discarded term placenta and have been reported to possess properties similar to both embryonic stem cells (ESCs) and MSCs [97]. Given that there are approximately 300,000 births each year in Australia alone, amnions provide an abundant potential source of regenerative cellular material and do not possess ethical constraints associated with sourcing ESCs from discarded embryos. Similar to ESCs, hAECs are pluripotent and have the ability to be expanded in culture [98], although the large number of cells that can be obtained from a single amnion may preclude the necessity for expansion in a clinical setting. Importantly and in contrast to ESCs, hAECs do not form teratomas in vivo [99, 100]. Significantly, hAECs display strong immunomodulatory and immunosuppressive properties and thus offer significant practical advantages for potential clinical applications in autoimmune disease therapy [95].
While stem cell therapy has shown promise in a number of disease models, the question of optimal administration is still unresolved and will most likely be disease-specific. However, the appropriate dosage and administration route are very important to the successful treatment of diseases with such therapies. With regards to stem cell therapy in neurodegenerative diseases, currently numerous pre-clinical studies have explored different administration routes, including local delivery into the CNS, intravenous and intraperitoneal injections. The rationale for local administration was to allow stem cells to engraft into the injured tissue and potentially differentiate into neuronal cells. Unfortunately, while some animal studies have demonstrated stem cell engraftment and potential neural differentiation, it is now widely believed that stem cells poorly engraft into the CNS tissue, despite their clinical effectiveness [101]. Moreover, clinical improvement has been found to be comparable following systemic or local stem cell delivery, which suggests that stem cells are most likely having their effect through the release of soluble factors [102]. Thus, the current consensus is that stem cell therapy is most likely acting by modulating the immune system and potentially increasing neuroregeneration, not through differentiation, but through the release of neurotrophic factors that support host neuroregeneration.
7 Immunomodulatory Capacity of hAECs
The immunomodulatory potential of hAECs has been studied in numerous in vitro assays and in vivo disease models. hAECs are believed to exert their immunomodulatory functions on several immune cells including T cells, B cells, and macrophages (Fig. 21.2). One of the mechanisms suggested is through the secretion of immunomodulatory factors. As shown by Li et al., conditioned media from hAEC cultures has the ability to inhibit cells of both the humoral and cell-mediated adaptive immune system, as shown by the reduction in T- and B-cell proliferation [103]. Various soluble factors have been implicated; these include alpha-fetoprotein (AFP), macrophage inhibitory factor (MIF), Fas ligand, TNF-related apoptosis-inducing ligand (TRAIL), TGF-β and human leukocyte antigen-G (HLA-G) [103] (Fig. 21.2). Studies have revealed that hAECs produce AFP [104], which has been shown to reduce lymphocyte activity and decrease neuroinflammation in an animal model of MS [105]. Additionally, hAECs express HLA-G on their surface and in soluble form [106]. HLA-G is a non-classical HLA class I antigen that is believed to provide protection for the developing fetus against the maternal immune system [107]. HLA-G has also been shown to induce apoptosis of activated CD8+ T cells and inhibits the proliferation of CD4+ T cells [108]. Furthermore, in vitro co-cultures have shown that hAECs can significantly suppress antigen-specific, allogeneic, and mitogen-stimulated T-cell responses [109, 110].


Fig. 21.2
Immunomodulatory properties of hAECs. hAECs are known to suppress the function of many immune cell types. hAECs can suppress T-cell activation, infiltration into inflamed tissue and can increase the number of anti-inflammatory Treg cells. hAECs can also decrease macrophage infiltration into inflamed areas and promotes polarization of macrophages to a pro-reparative M2 phenotype. These immunomodulatory actions are believed to be due to the release of soluble factors including PGE2, TGF-β, Fas-L, AFP, MIF, TRAIL, and HLA-G. hAECs have been shown to modulate the host immune system in vivo, by decreasing the production of TNF-α, IFN-γ, MCP-1 and IL-6 and increasing the production of anti-inflammatory cytokines IL-10 and IL-4
Currently available data suggest that the immunomodulatory and reparative actions of hAECs are intrinsically linked with macrophage activity. In an inflammatory lung injury model, where damage was induced by administration of bleomycin, a potent stimulator of lung fibrosis, hAECs were found to modulate the host inflammatory response, reduced lung fibrosis, and prevented the loss of lung function [111]. A subsequent study was performed using surfactant protein C knockout mice, which are highly susceptible to lung injury due to impaired macrophage function. It was found that following the bleomycin insult, administration of hAECs was unable to decrease lung fibrosis or improve lung function [112]. The authors postulated that this failure of hAECs to exert their reparative effect was directly due to impairment of the normal host macrophage function. Furthermore, hAECs were shown to directly influence macrophage behavior, in vitro and in vivo, and polarized macrophages toward a pro-reparative M2 phenotype [113] and this polarization was suggested to be critical for the hAECs reparative effect observed in this lung fibrosis model. Similarly, in a model of liver fibrosis [114], transplanted hAECs have been associated with polarization of macrophages to an M2 phenotype associated with an increase in IL-10 and reduction in fibrosis. Macrophages are understood to have a dual role in the pathophysiology of MS. In active MS lesions associated with high levels of pro-inflammatory cytokines, classically activated or M1 macrophages mediate significant cell injury. However, an alternatively activated M2 type of macrophage can be generated through exposure to cytokines such as IL-4 and IL-10 and are involved with cell repair [115]. Although the influence of hAEC on the macrophage polarization in EAE or MS has not been directly examined, macrophage infiltration has been shown to be reduced in chronic EAE [116]. Moreover, studies in spinal cord transection [117] and intracerebral hemorrhage [118] have found reduced gliosis and microglia in animals treated with hAEC.
In addition, hAEC administration has been shown to directly suppress the host immune response. In the bleomycin lung fibrosis model, hAEC administration significantly decreased the local pro-inflammatory response in the lungs by decreasing the expression of TNF-α and IFN-γ [111]. Interestingly, hAEC treatment also significantly reduced the expression of IL-6 and TGF-β [111], both of which are known to drive the differentiation of pro-inflammatory Th17 cells [49], which play an important role in EAE and MS pathogenesis. In a fetal sheep model of intrauterine inflammation, hAECs were found to attenuate local lung inflammation with a decrease in TNF-α, IL-1β, and IL-6 observed [119]. Moreover, hAECs reduced local brain inflammation and subsequently decreased white and grey matter injury [120]. Emerging data from our lab [109] and others [116] have also suggested that hAECs can modulate the autoimmune response in EAE, an animal model of MS. It was reported that intravenously administered hAECs reduced immune cell infiltration into the CNS and consequently demyelination was reduced in these animals. Furthermore, the authors postulated that this effect was due to a Th2 bias that may in part be mediated by TGF-β and PGE2 [116]. The exact mechanisms and contribution of other immune cells to this observed immunomodulation is yet to be elucidated, however results thus far are promising and warrant further investigation.
8 Neuroprotective/Regenerative Ability of hAECs
Stem cells have the capacity to work by means of paracrine neuroprotective, angiogenic and anti-inflammatory mechanisms, along with the expression of neurotrophic and survival factors that have the potential to stimulate endogenous progenitor cells [121]. Given the lack of sufficient endogenous repair within the CNS there is tremendous interest in cell therapies with stem cell-like properties that could potentially replace and repair injury to the CNS. hAECs are formed from aminoblasts derived from the embryonic epiblast, 8 days after fertilization and prior to gastrulation and thus share many features of ESCs. Moreover, hAECs have been found to express characteristic ESC genes including POU5F1, SOX2, CFC1, NANOG, DPPA3, PROM1, and PAX6 and surface antigens SSEA-4 and GCTM2 [99], suggesting potential for multipotency. Furthermore, mRNA expression and positive immunocytology evidence of immature and mature neural antigens such as nestin and GFAP in hAECs have suggested that these cells are predisposed to differentiate into mature neural cells. In fact, high-density cultures consisting of hAEC spheroid structures have been compared with neurospheres due to the heterogeneity of immature neuronal and glial cell surface protein expression [122, 123]. Following culture in neural differentiating medium, most hAECs appear to express GFAP with astrocyte morphology [99] while a minority express NES or MAP2 neuronal markers.
In vivo studies using hAECs have demonstrated cells can survive and function for at least 4 weeks, some studies up to 8 weeks, following xenogenic transplantation with no evidence of recognition or rejection by the host immune system [124]. A number of studies have demonstrated the therapeutic efficacy of hAEC administration in different neurodegenerative diseases (summarized in Table 21.1). Furthermore, these studies have shown hAECs possess differentiation potential in vivo with the expression of neural markers nestin, MAP2 and GFAP after transplantation of hAECs into rodents [118, 125, 126]. AECs have also been isolated from rats and shown to survive and express MAP2 in a gerbil cerebral ischemia model [127]. Furthermore, significant repair, remyelination and improved spinal cord electrophysiology was observed in a rat spinal cord injury model following transplantation of hAEC within a muscle graft [128]. However, the authors could not identify any neuronal differentiation to account for these findings. In another neurodegenerative model, Kakishita et al. [129] found improved apomorphine-induced rotational asymmetry in the dopamine-depleted striatum of immunosuppressed rats as a result of hAEC transplantation, but found the cells retained their round or oval morphology rather than obtaining neuronal morphology.
Table 21.1
Pre-clinical studies investigating the therapeutic efficacy of hAECs in neurological disorders
Neurological disorder | Study outcome | References |
---|---|---|
Parkinson’s disease | hAECs decreased rotational asymmetry and grafts expressed nestin and vimentin | Yang et al. [126] |
Spinal cord injury | hAECs promoted growth and sprouting of host axons and prevented glial scar formation | Sankar et al. [117] |
Overexpression of growth factors in hAECs improved NSCs survival and differentiation in vitro and promoted NSCs differentiation in vivo | Meng et al. [131] | |
hAECs promoted regeneration and sprouting of axons and improved hindlimb motor function | Wu et al. [130] | |
Stroke | hAECs expressed vimentin and nestin in vivo, reduced activated microglia at the injury site and brain edema and improved the motor deficits | Dong et al. [118] |
hAECs were readily transduced with lentiviral vectors. hAECs administration rescued the neurological deficits and decreased neuronal cell death and reduced lesion volume | Liu et al. [125] | |
Multiple sclerosis | hAECs potently attenuated disease, reduced T-cell and macrophage infiltration and reduced demyelination | Liu et al. [116] |
Neonatal brain injury | hAECs reduced CNS inflammation and neuronal cell death | Yawno et al. [120] |
hAEC transplantation has been associated with improved clinical recovery in a number of CNS injury models including intracerebral hemorrhage [118], cerebral ischemia [125], spinal cord transection [130, 131], and MPTP and 6-OHDA-induced toxicity. These effects do not appear to be explained solely by the differentiation of hAECs into neurons, but instead suggest the hAECs produce neurotrophic factors that may protect neurons and promote endogenous repair. This observation is supported by in vitro work that clearly demonstrates that the conditioned media of hAECs can improve neuron survival and increase neurite outgrowth [132]. AECs are known to produce a number of different growth factors with the potential to facilitate neuroregeneration. Uchida et al. [132] demonstrated that hAEC produce BDNF and NT3 and that these factors were partially responsible for the improved neuron survival and neurite outgrowth in embryonic rat neuronal cell culture. Similarly, BDNF and NT-3 were identified in the conditioned media of hAEC cultures and it was shown that these factors could improve neural stem cell survival [133]. However, these specific factors alone were not as effective as total hAEC-conditioned media, suggesting that other important factors are yet to be identified.
Sakuragawa et al. [134] observed an increase in survival of E18 cortical neurons with hAEC-conditioned media and concluded that the responsible neurotrophic factor is unknown but may have properties similar to EGF since this cell type only responds to EGF but hAEC did not appear to express EGF mRNA. Correspondingly, Venkatachalam et al. found that hAEC-conditioned media improved the survival and differentiation of chicken neural retinal cells which are known to be highly responsive to FGF-2 and therefore the authors concluded that the responsible neurotrophic factor from the conditioned media was possibly related to FGF-2 [135]. However, examination of hAEC-conditioned media for known isoforms of EGF and FGF-2 by Western blot was negative. Recently, the neurotrophic factor pleiotrophin was identified in the conditioned media of hAECs, and furthermore pleiotrophin was demonstrated to promote the differentiation of UCB-MSC into dopaminergic (DA) neurons in vitro [136]. Multiple roles for pleiotrophin in neurite outgrowth and migration, repair and differentiation of progenitor cells have been described [137, 138]. It is likely that the neurotrophic effect of hAECs is the result of multiple growth factors acting synergistically rather than in isolation. hAECs have also been shown to produce catecholamines including dopamine and norepinephrine through tyrosine hydroxylase [139]. This discovery led to a large number of studies investigating the beneficial role of hAEC transplantation in animal models of Parkinson’s disease, a neurodegenerative condition characterized by loss of DA neurons. Collectively these studies demonstrated improved survival of DA neurons associated with improved clinical scores in MPTP and 6-OHDA rat models, following intracerebral hAEC transplantation [123, 126, 129, 140]. The mechanism for these observations remains controversial. While hAECs can differentiate into DA neurons in vitro, the observed in vivo improvements appear greater than would be expected given the limited survival of hAEC-derived DA neurons [129]. The production of neurotrophic factors is a more plausible explanation, which is supported by improved survival of DA neurons when cultured in hAEC-conditioned media [141]. Although high doses of catecholamines are clearly neurotoxic, low doses have been shown to protect neurons against free radical-induced oxidative stress [142]. Furthermore, Noh et al. also demonstrated a synergistic action of dopamine in prolonging the neuroprotective effects of BDNF on neuronal cell death [142].
9 Conclusions
Given the lack of successful treatments available for many relapsing–remitting and progressive MS patients, there is an urgent need to develop therapies that can treat the multitude of pathologies that are involved in MS. As outlined in this review the involvement of the immune system is very important and has thus far been the primary target for current clinical treatments. However, as we have highlighted, neurodegeneration plays an equally important role in disease pathogenesis, particularly in the progressive stages. Furthermore, these two pathways do not act independently and by minimizing peripheral and central (directly within the CNS) inflammation, this can indirectly protect the CNS from neurodegeneration. Therefore, future treatments need to address both of these components. As highlighted in Table 21.2, stem cells, particularly hAECs, offer the ability to target and reverse multiple pathological pathways that contribute to the development and progression of MS. For instance, pathological consequences of MS include increased T-cell activation, increased generation of pathogenic Th1 and Th17 cells and little or no neuroregeneration. Conversely, hAECs have been shown to inhibit T-cell activation, suppress the development and pro-inflammatory actions of Th1 and Th17 cells and in addition can secrete neurotrophic factors such as BDNF, NGF, and pleiotrophin that promote neuroregeneration.
Table 21.2
Potential of hAECs to reverse pathological consequences of MS
Pathological consequences of MS | Beneficial effects of hAECs |
---|---|
Increased T-cell activation | Inhibits T-cell activation |
Increased number of Th1/Th17 cells | Suppresses actions of Th1/Th17 cells |
Decreased number of Th2/Treg cells | Promotes generation of Th2/Treg cells |
Little/no neuroregeneration | Secrete neurotrophic factors to aid in neuroregeneration (e.g. BDNF, NGF, pleiotrophin) |
Formation of glial scar tissue | Promotes M2 macrophage polarization which aids in tissue remodeling and decreases fibrosis |
While hAECs promise to be an adjunct for the treatment of inflammatory and neurodegenerative diseases, an important question that still remains is their safety once transplanted into patients. Studies have shown that hAECs are immunoprivileged and immunosuppressive and therefore appear to be safe to use in an allogeneic setting. However, the requirement of HLA matching is still to be determined. In this context it is worth noting that amniotic membrane and its epithelial layer (from which these hAECs originate) have been used for over 30 years to treat several clinical conditions [143]. These include: dural defects [144], ocular surface reconstruction in conditions such as keratopathy [145] and conjunctival Bowens disease [146], as well as for the treatment of burn lesions [147]. In these conditions, hAECs have been shown to be well tolerated, did not form tumors and did not result in graft rejection. Given the current safety profile of the amnion, it is likely that hAECs could be investigated in phase I clinical trials. However, issues associated with their immunogenicity and other factors will need to be carefully assessed before trials are commenced. This aspect is particularly pertinent, given that hAEC graft survival can be significantly reduced in pre-immunized animals [148]. This was illustrated in a study by Gabler and Lohmann, when serial transplantation of amniotic membrane into the eye (which is immune privileged) generated an inflammatory response after the second and third transplantation [149]. These results suggest that the continuous immunosuppressive and regenerative effects of hAECs in a transplantation setting will require several immunological barriers to be overcome.
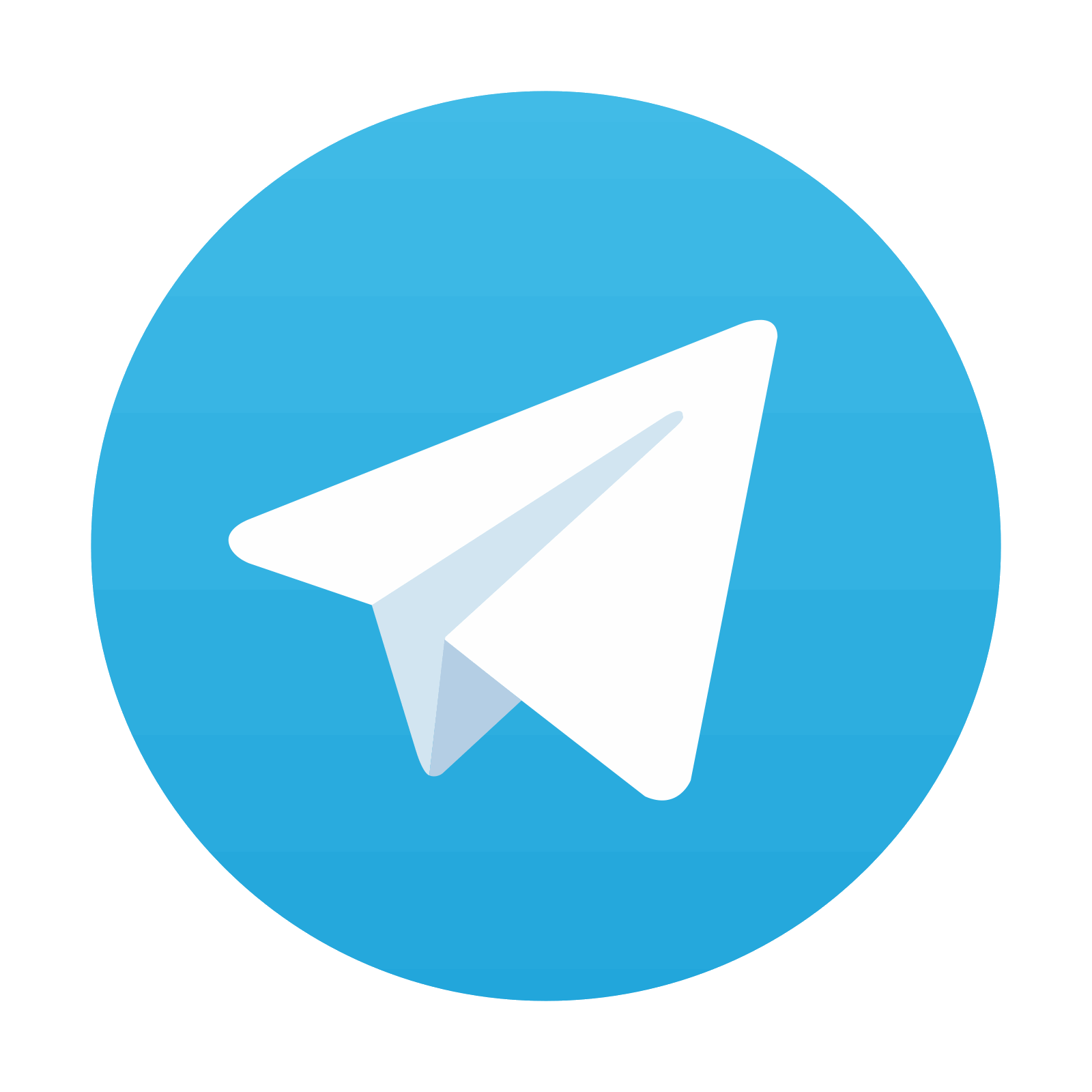
Stay updated, free articles. Join our Telegram channel
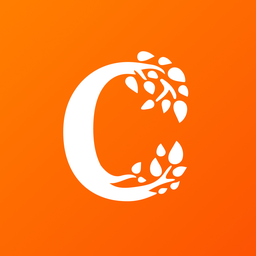
Full access? Get Clinical Tree
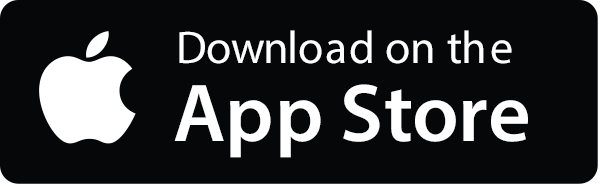
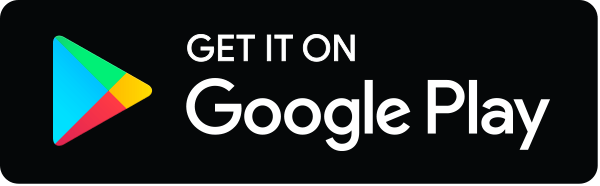