Chapter 4 The kidneys
Introduction
The kidneys have three major functions:
The kidneys have a rich blood supply and normally receive about 25% of the cardiac output. Most of this is distributed initially to the capillary tufts of the glomeruli, which act as high pressure filters. Blood is separated from the lumen of the nephron by three layers: the capillary endothelial cells, the basement membrane and the epithelial cells of the nephron (Fig. 4.1). The endothelial and epithelial cells are in intimate contact with the basement membrane; the endothelial cells are fenestrated, and contact between the epithelial cells and the membrane is discontinuous so that the membrane is exposed to blood on one side and to the lumen of the nephron on the other.
Medullary hyperosmolality, which is vital for the further reabsorption of water, is generated by the counter-current system, summarized in Figure 4.2. Chloride ions, accompanied by sodium, are pumped out of the ascending limbs of the loops of Henle into the surrounding interstitial fluid, and thence diffuse into the descending limbs. As the ascending limbs of the loops of Henle are impermeable to water, the net effect is an exchange of sodium and chloride ions between the ascending and descending limbs. This alters the osmolality of both the fluid within the nephrons and the surrounding interstitial fluid. A gradient of osmolality is set up between the isotonic corticomedullary junction and the extremely hypertonic (approximately 1200 mmol/L) deep medulla. Diffusion of urea from the collecting ducts into the interstitium and thence into the loops of Henle also makes an important contribution to medullary hypertonicity. It is noteworthy that urinary concentrating ability is impaired in malnourished children but can be restored by increasing their dietary protein intake or (experimentally) by adding urea to their diets.
Approximately 90% of the filtered sodium and 80% of the filtered water has been reabsorbed from the glomerular filtrate by the time it reaches the beginning of the distal convoluted tubules. In the distal tubules, further sodium reabsorption takes place, in part controlled by aldosterone; this generates an electrochemical gradient that is balanced by the secretion of potassium and hydrogen ions. Ammonia is also secreted in the distal tubule and buffers hydrogen ions, being excreted as ammonium ions (see p. 43).
The Biochemical Investigation of Renal Function
Measurement of glomerular filtration rate
Clearance
U = urinary creatinine concentration (µmol/L)
= urine flow rate (mL/min or (L/24 h)/1.44)
P = plasma creatinine concentration (µmol/L)
There are two main alternative approaches to determining the GFR in clinical practice. These are to use exogenous markers of clearance or to derive an estimated GFR (eGFR) from the plasma creatinine concentration (see p. 67). GFR can be measured by measuring the disappearance from the blood of a test substance that is completely filtered by the glomeruli and neither secreted nor reabsorbed by the tubules, following a single injection. This approach has the advantage that a urine collection is not required. Suitable substances for this purpose include 51Cr-labelled EDTA (ethylenediaminetetra-acetic acid), 125I-iothalamate (for which the decline in plasma radioactivity is monitored) and iohexol, a non-radioactive X-ray contrast medium that is simple to measure using high performance liquid chromatography. Typically, blood samples are taken at 2, 3 and 4 h after injection, although samples taken over a longer period may be required in renal impairment.
Plasma creatinine
The reference range for plasma creatinine in the adult population is 60–120 µmol/L, but the day-to-day variation in an individual is much less than this range. Equation 4.1 indicates that plasma creatinine concentration is inversely related to the GFR. GFR can decrease by 50% before plasma creatinine concentration rises beyond the normal range; plasma creatinine concentration doubles for each further 50% fall in GFR. Consequently, a normal plasma creatinine does not necessarily imply normal renal function, although a raised creatinine does usually indicate impaired renal function (Fig. 4.3). Furthermore, a change in creatinine concentration, provided that it is outside the limits of normal biological and analytical variation, does suggest a change in GFR, even if both values are within the population reference range (see Case history 1.2).
Estimated GFR
where [sCr] = serum creatinine concentration (µmol/L) and age is measured in years. This formula is for white males: the result should be multiplied by 0.742 for females and by 1.21 for African Caribbean people. (A calculator is available at: www.renal.org.)
A six-variable formula includes serum urea and albumin concentrations in addition. Because different laboratories may measure creatinine using different techniques, correction factors can be used to relate the calculated eGFR to the reference method for creatinine measurement, thus supposedly ensuring that all eGFR values are comparable. However, although the recommended formula for routine reporting in UK laboratories is the four-variable MDRD formula, it should be appreciated that it was derived from studies on patients with chronic kidney disease and may not be applicable to subjects with normal or near-normal renal function. Indeed, it is recommended that values of eGFR greater than 60 mL/min should be reported as ‘>60 mL/min’ and regarded as normal in the absence of clinical or laboratory evidence of renal disease (e.g. abnormalities on imaging, proteinuria or haematuria). Neither is it applicable in acute kidney disease, pregnancy, in conditions in which there is severe muscle wasting, oedematous conditions, amputees or in children. A major use of the MDRD eGFR is as a tool for screening for chronic kidney disease (CKD). UK guidelines recommend annual screening using eGFR and urinary protein in patients at risk of developing CKD, as summarized in Fig. 4.4. A more recent formula, the CKD-EPI (Chronic Kidney Disease Epidemiology Collaboration), is based on pooled data from several studies and correlates better with measured GFR than the original MDRD formula, especially at values above 60 mL/min.
Plasma urea
Factors affecting the ratio of plasma urea to creatinine are summarized in Figure 4.5. Changes in plasma urea concentration are a feature of renal impairment, but it is important to consider possible extra-renal influences on urea concentrations before ascribing any changes to an alteration in renal function.
Assessment of glomerular integrity
Impairment of glomerular integrity results in the filtration of large molecules that are normally retained and is manifest as proteinuria. Proteinuria can, however, occur for other reasons (see p. 79). Clinical proteinuria is proteinuria that can be reliably detected by dip-stick testing of urine, and is >300 mg/L. The significance of microalbuminuria (increased urinary albumin excretion, but not to an extent that can be detected by conventional dip-sticks) is considered in Chapter 11.
Renal Disorders
Many disorders primarily affect renal tubular function, but most are rare. Their metabolic and clinical consequences range from being trivial (as in isolated renal glycosuria) to being serious (as in cystinuria, see p. 82).
Acute kidney injury
AKI is conventionally divided into three categories, according to whether renal functional impairment is related to a decrease in renal blood flow (pre-renal), to intrinsic damage to the kidneys (intrinsic), or to urinary tract obstruction (post-renal). Should any of these occur in a patient whose renal function is already impaired, the consequences are likely to be more serious. Some clues to the presence of chronic disease in a patient with AKI (‘acute on chronic’ kidney injury) are discussed in Case history 4.3.
Pre-renal acute kidney injury
Pre-renal uraemia is essentially the result of a normal physiological response to hypovolaemia or a fall in blood pressure. Stimulation of the renin–angiotensin–aldosterone system and vasopressin secretion typically results in the production of a small volume of highly concentrated urine with a low sodium concentration (a fact that may be helpful in distinguishing between pre-renal and intrinsic AKI; Case history 4.1; Fig. 4.6). Renal tubular function is normal, but the decreased GFR results in the retention of substances normally excreted by filtration, such as urea and creatinine. Decreased excretion of hydrogen and potassium ions results in a tendency to metabolic acidosis and hyperkalaemia (the latter often being exacerbated by tissue damage).
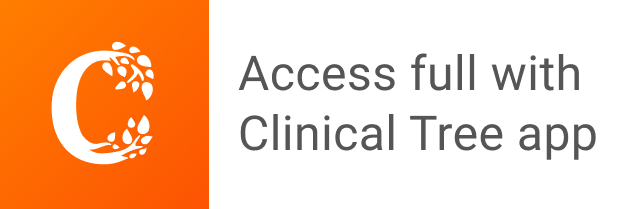