and Miguel A. R. B. Castanho2
(1)
Instituto de Bioquímica Médica Leopoldo de Meis, Federal University of Rio de Janeiro, Rio de Janeiro, Rio de Janeiro, Brazil
(2)
Institute of Biochemistry and Institute of Molecular Medicine, School of Medicine, University of Lisbon, Lisbon, Portugal
Diversity is essential to the sustainability of living systems. This is true for species in ecosystems as it is for molecules in cells, tissues, and organisms. Yet, the same way different species are linked by common ancestors and may be grouped in taxonomic classes according to common characteristics they share, molecules may be grouped in classes and classified according to common chemical and physical characteristics. One of such characteristics is solubility in water (in other words: how polar atoms are distributed in the 3D structure of molecules). One class of biological molecules, the lipids , includes only low water solubility (“hydrophobic”) molecules, this being the characteristic that defines this class. Other classes include molecules that are mostly moderately or highly soluble in water and can be recognized for the dominant presence of specific chemical groups: OH in saccharides (also referred to as “carbohydrates”) and a combination of amino and carboxyl groups in amino acid s . Lipids, saccharides, and amino acids may combine with molecules of their own class to form either polymers (molecules formed by successively covalently attaching smaller molecules), such as polysaccharides and proteins , or supramolecular assemblies (organized arrangements of molecules that are in contact but are not covalently attached), such as the lipid bilayer of cell membranes . It is common to find molecules and supramolecular assemblies that combine elements from different classes, such as nucleotides , which contain saccharides. Proteins are extremely versatile in this regard because protein interactions with saccharides, lipids, and nucleic acids (nucleotide polymers) are ubiquitous in virtually all cells.
Figure 3.1 depicts the basic principles that support the organization of biological molecules in different classes.
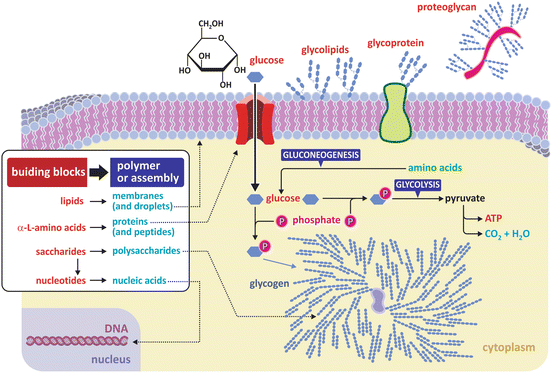
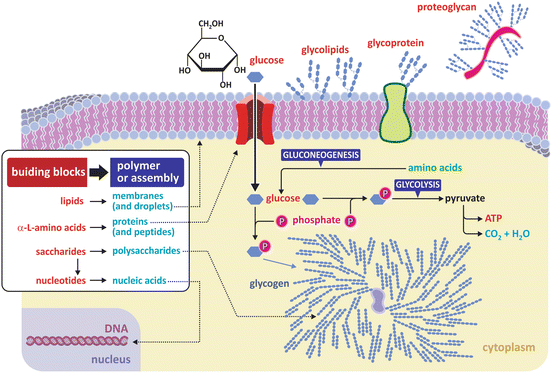
Fig. 3.1
Three classes of fundamental molecules (“building blocks”) are enough to organize in different families most biological macromolecules (polymers) and large supramolecular assemblies (such as lipid bilayers and lipid droplets). Nucleic acids are polymers of nucleotides , which have saccharide residues in their composition. Proteins are polymers that are commonly combined with molecular residues of other classes such as saccharides (glycoproteins or proteoglycans when the saccharidic content is high). Glycolipids (glycosylated lipids —lipids with saccharide groups attached) are also common in membranes . Both building blocks and polymers/assemblies are important in the structure and functioning of cells, constituting the vast majority of matter in a cell (excluding water). Glycogen is a branched saccharide polymer (polysaccharide). Branching occurs at every 10 glucose monomer residues approximatly (branching unrealisticly highlighted in the figure for illustrative purposes)
3.1 Lipids and the Organization of Their Supramolecular Assemblies
Lipids are highly hydrophobic molecules that nonetheless may have polar chemical groups in their composition. Because one portion of the structure of the molecule is polar and the other is non-polar, the molecule is referred to as amphiphilic, which stresses its dual nature: the polar part will tend to interact with water and other polar molecules, and the other will tend to minimize its interaction with water and other polar molecules. Nevertheless, it is important to bear in mind that lipids are molecules in which hydrophobicity predominates, even if they are amphiphilic. This is a qualitative definition with no clear boundaries in terms of molecular structure, which is nonetheless a useful working definition because hydrophobicity grants lipids the ability to organize in supramolecular assemblies that are very distinctive from polar molecules. Take lipid bilayers as example: they are very extensively organized supramolecular assemblies that are very stable and yet do not involve covalent bonds between lipid molecules. Lipids spontaneously self-associate in aqueous environments, and amphiphilic lipids in particular may self-associate in a very organized way. This results from the so-called hydrophobic effect , although the most appropriate term would be “entropic effect.”
The entropic effect is a corollary of the second law of thermodynamics, which in one of its possible statements implies that all physical and chemical events tend to evolve in a way so that total entropy (“disorder”) increases. Consider Fig. 3.2; strongly amphiphilic molecules of generic cylindrical or rectangular cuboid shape will spontaneously form a bilayer to minimize the contact of nonpolar regions with water. The driving force for this event may be counterintuitive at first glance: the bilayer is the arrangement that corresponds to the most disordered system. This may seem absurd because we tend to focus our attention in the solute (the lipids , in this case) and forget the solvent (water); yet, the gain in entropy refers to both. The lipids become ordered relative to each other, but the contact of hydrophobic groups with water molecules imposes restrictions to the orientational freedom of water, which is very costly in terms of entropy.
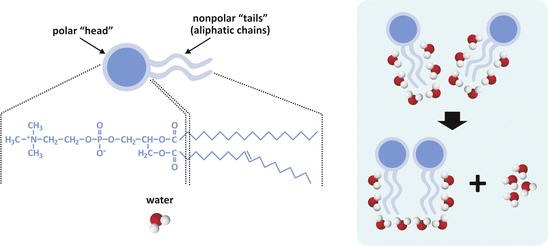
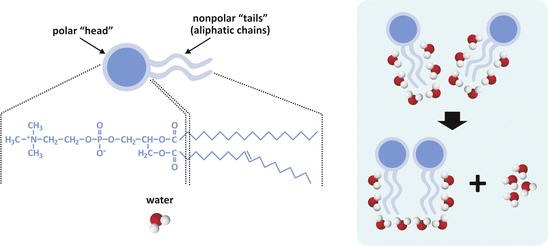
Fig. 3.2
Simplistic representation of two molecules of lipids having two aliphatic (hydrocarbon) chains and a polar “head.” Polar heads usually contain phosphate (phospholipids ) and other polar groups (left panel). Nonpolar regions of lipids tend to associate to each other because fewer molecules of water get exposed to the aliphatic chains (right panel). Exposed aliphatic chains force molecules to orient their oxygen atom away from the hydrocarbon constituents, i.e., they force a certain degree of order to the solvent. When two lipid molecules associate, less water molecules are forced to order and although the lipid molecules become more ordered relative to each other, the whole molecular systems (water included) becomes more disordered, in agreement with the entropic formulation of the second law of thermodynamics. Thus, this is named the entropic effect (occasionally imprecisely referred to as “hydrophobic effect ”). The same principle applies when 3, 4, 5, …, n molecules form large assemblies of lipids, such as lipid bilayers (see Sect. 3.1.1)
Broadly speaking, diacyl lipids (i.e., lipids containing two acyl—aliphatic—chains) with polar “heads”, such as most of the lipids found in cell membranes , have a generic shape with the characteristics depicted in Fig. 3.2, and the entropic effect compels these molecules to an ordered and parallel self-association. This is the physical process that sustains the stability of cell membranes as we shall see in Sect. 3.1.1. Now we will look more closely into the chemical structure of diacyl lipids: although these lipids tend to assemble as bilayers, the differences in their chemical nature determine which chemical processes they are able to participate in (e.g., cell signaling ) and their tendency to associate to membrane proteins and/or form specific domains in the membrane. Most triacyl lipids occurring in nature, such as triacylglycerols (often referred to as triglycerides), lack a bulk polar “head” group and therefore do not display the propensity to form ordered supramolecular structures such as membranes (Fig. 3.3). Instead, they self-associate disorderly, forming aggregates, such as the lipid droplets found in cells at the site these lipids are synthesized (hepatocytes ) or stored (adipocytes). Figure 3.3 shows histological images of the adipose tissue in which the presence of lipid aggregates occupying almost all cellular volume is detected.
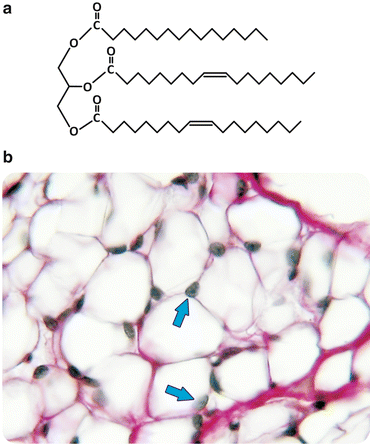
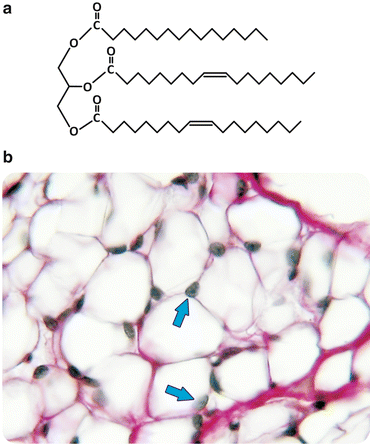
Fig. 3.3
Triacylglycerols lack bulk polar “head” groups, which keeps them from self-assembly into bilayers (a). Triacylglycerols aggregate in disordered agglomerated structures that can be detected in histological preparations of adipocytes (b). In panel (b) the lipids were extracted leaving white empty areas; arrows point to the nucleus. Figure reprinted with the permission of Instituto de Histologia e Biologia do Desenvolvimento, Faculdade de Medicina, Universidade de Lisboa, FMUL
Fatty acids can be considered as the basic unit common to most lipids in human cells. They consist of a linear unbranched aliphatic chain (“tail”) with a carboxyl group (Fig. 3.4a). The aliphatic chain can be saturated (i.e., having all the carbon–carbon bonds reduced to simple bonds, without double or triple bonds) or unsaturated. Most fatty acid s in humans have an even number of carbons, ranging from 10 to 28. Fatty acids are important molecules in the energetic metabolism of the organism, and they can be found either in “free” form (unattached to other molecules) or attached to other molecular structures through ester bonds (Fig. 3.4d, e). Free fatty acids differ from each other in the number and position of double bonds they have and in the length (in practice, the number of carbons) of the chains. The diversity attainable by changing these characteristics is virtually infinite, but, in practice, not all combinations are detected in nature (Table 3.1 displays examples of the most frequent fatty acids found in the human cells). Naturally, the nomenclature of fatty acids highlights their characteristic differences in terms of number and position of double bonds and chain length (Fig. 3.4b). The most recent nomenclature systems are very descriptive of these characteristics, although older, traditional nomenclature systems are widely used. The recent nomenclature identifies the carbon atoms in a chain by number, starting consecutively from the carbon of the carboxyl group (see Fig. 3.4b). Double bonds, if existent, are identified by the number of the carbon of lowest order forming the bond. The ω, or n, nomenclature numbers the carbons in reverse order (see Fig. 3.4b). Another nomenclature, still popular among many biochemists, identifies carbons by Greek letters in alphabetical order (α, β, γ, δ…) starting from the carbon adjacent to the carboxyl group (see Fig. 3.4b). A process of metabolic degradation of lipids is titled “β-oxidation ” because of the importance of carbon β.
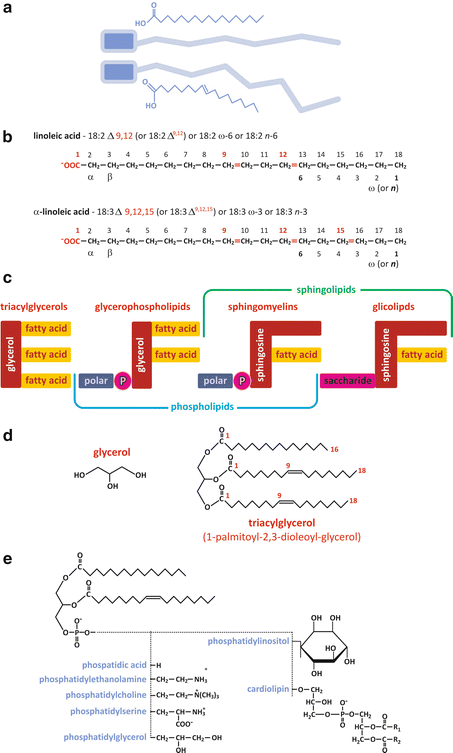
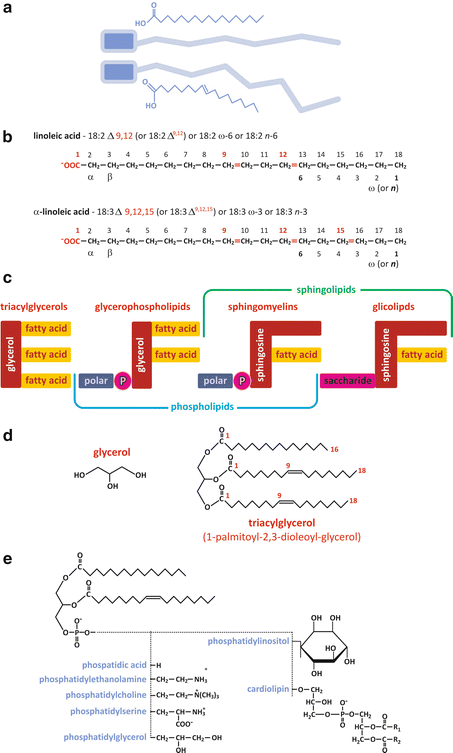
Fig. 3.4
(a) Pictoric representation of free fatty acid s , highlighting the polar carboxylic group in blue and the nonpolar aliphatic chain in gray; double bonds impose constraints in the conformation of the chain, preventing stretching and rotation around the carbon skeleton, for instance. (b) Illustrative examples of the application of different nomenclature systems. Recent systems (numbering carbons from the carboxylic group) are more precise and descriptive, but older systems (Greek lettering and ω—numbering from the non-carboxylic ending carbon) are still widely used in some disciplines as nutrition. Trivial (nonsystematic) names (Table 3.1) were never abandoned for their “user-friendly” nature. (c) Fatty acids form ester bonds with other molecular structures to form more complex lipids such as triacylglycerols [detailed example in panel (d)], glycerophospholipids, or sphingolipids. Fatty acid residues are present in all. In sphingomyelins the polar group attached to phosphate is either choline or ethanolamine [panel (e)]. The example of specific triacylglycerol in (d) is 1-palmitoyl-2,3-dioleyl-glycerol . (e) Glycerophospholipids form different classes depending on the chemical nature of the “polar head” (fatty acid chains in this example are illustrative)
Table 3.1
Nomenclature of the most common fatty acid s found in humans, both saturated (only simple carbon–carbon bonds) and unsaturated
Number of C (chain length) | Saturated | Unsaturated | Number double bonds | Nomenclature system | ||
---|---|---|---|---|---|---|
Δ | ω | n | ||||
14 | Myristic | |||||
16 | Palmitic | Palmitoleic | 1 | 16:1 Δ9 | 16:1 ω − 7 | 16:1 n − 7 |
18 | Stearic | Oleic | 1 | 18:1 Δ9 | 18:1 ω − 9 | 18:1 n − 9 |
Linoleic | 2 | 18:2 Δ9,12 | 18:2 ω − 6 | 18:2 n − 6 | ||
α-Linoleic | 3 | 18:3 Δ9,12,15 | 18:3 ω − 3 | 18:3 n − 3 | ||
γ-Linoleic | 3 | 18:3 Δ6,9,12 | 18:3 ω − 6 | 18:3 n − 6 | ||
20 | Arachidic | Arachidonic | 4 | 18:4 Δ5,8,11,14 | 18:4 ω − 6 | 18:4 n − 6 |
24 | Lignoceric |
Glycerolipids such as diacylglycerols and triacylglycerols result from the chemical conjugation of a glycerol molecule with fatty acid s through sterification (Fig. 3.4). Yet, glycerolipids may also conjugate other groups besides fatty acids. Usually a phosphate group is used to bridge glycerol to a polar group, forming glycerophospholipids (Fig. 3.4). The phosphate binds both the glycerol and the polar group through phosphodiester bonds, which are ubiquitous in biochemical processes for their importance in molecular structure and energetics.
Glycerophospholipids are named according to the fatty acid s attached to glycerol and the chemical nature of the polar group (Fig. 3.4e).
Sphingolipids constitute another class of lipids present in many human cells, being responsible for rigid domains in the membranes . Figure 3.5 shows that although these lipids are similar in structure to glycerophospholipids, they are chemically different. These differences are responsible for the formation of rigid bilayers when mixed with phospholipids and cholesterol .
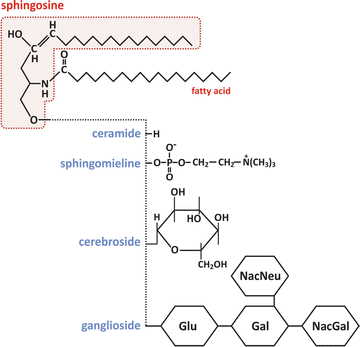
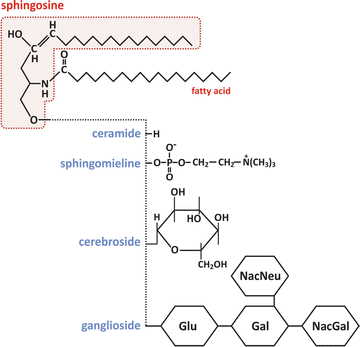
Fig. 3.5
Molecular structure of sphingolipids. As with glycerophospholipids, sphingolipids form different classes depending on the chemical nature of the “polar head” (the fatty acid chain in this example is illustrative). Glu – glucose, Gal – galactose, NacGal – N-acetylgalactosamine, NacNeu – N-acetylneuraminic acid
Sterols, of which cholesterol in an example (Fig. 3.6), are extremely hydrophobic alcohols. These molecules are characterized by a flat rigid system of carbon rings, cyclopentaphenanthrene, and have some remarkable properties:
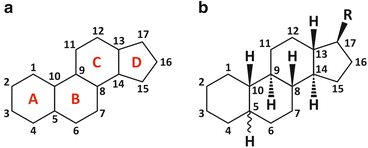
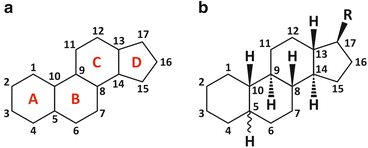
Fig. 3.6
Cyclopentaphenanthrene [panel (a)] is the basic structure of steroids [panel (b)], in which R is a generic group. The numbering system of carbons is highlighted. Cyclopentaphenanthrene is extremely rigid, planar, and hydrophobic. Sterols are a subfamily of steroids having an alcohol group in carbon 3 (see Table 3.2). Sterols can be found in the lipid membranes of animals, fungi, and plants but not bacteria. Sterols are the targets of some anti-infectious drugs in humans (this issue is readdressed in Fig. 3.9)
1.
Only one small polar group present (OH in cholesterol ; C=O in the equivalent ketones).
2.
A very flat molecule formed of four coplanar rings.
3.
When placed in lipid bilayers, it acts as a fluidity regulator (not too rigid to compromise dynamics and not too fluid to compromise barrier integrity).
Cholesterol is a vital molecule to humans (Box 3.1). In addition to its properties in membranes , it takes part in other biochemical phenomena such as the synthesis of some hormones. Nevertheless, the seriousness of problems associated to its ingestion in excessive amounts in unhealthy diets, associated to its very low solubility in aqueous media, turns this important molecule into a potential killer. Curiously, although cholesterol is essential to many species in nature, including humans (Table 3.2), its synthesis is relatively recent in evolutionary terms as it uses molecular oxygen , which was only present in atmosphere after the advent of photosynthesis.
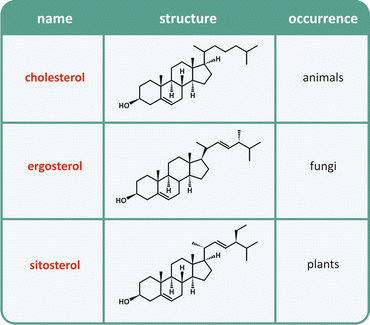
Table 3.2
Naturally occurring sterols
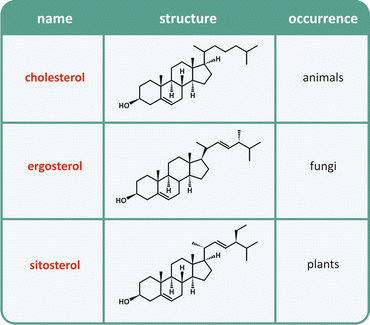
Box 3.1: Cholesterol: A Hero with Bad Reputation
High concentration (“level”) of cholesterol circulating in the blood is a major risk factor for cardiovascular disease, which affects a significant fraction of the whole population in many countries. The campaigns to prevent cardiovascular disease are often centered in reducing dietary cholesterol, which gives the impression that cholesterol is some kind of poison or toxin that should be banned. The persistent unhealthy doses of cholesterol in diet are indeed harmful, but this must not create the illusion that cholesterol is by itself harmful to cells. Virtually all molecules in excessive doses are harmful and cholesterol is not an exception. Moreover, human body is so dependent on cholesterol that it synthesizes its own cholesterol and has homeostatic mechanisms to regulate its production in connection with several metabolic processes.
The way the human body works at biochemical and physiological levels is crucially dependent on cholesterol . This extremely hydrophobic molecule intervenes in three major processes in humans:
Because of its hydrophobicity, the solubility in aqueous media such as blood is very low. There are specialized structures, lipoproteins, which incorporate cholesterol and form stable emulsions in aqueous medium. When cholesterol is not contained in these structures and/or lipoproteins deteriorate due to oxidation, depots are formed in the endothelial tissue that forms blood vessels. These depots are typical of atherosclerosis. In the most extreme cases, the blood vessels may be blocked and neighboring tissues are not irrigated. Lack of nutrients and oxygen (ischemia) may cause severe lesions in tissues. Vulnerable atherosclerotic plaques may also detach and clog other vessels. Any of these conditions is called infarction. Myocardial infarction, commonly known as a heart attack, is one example. Cerebral infarction is another example, commonly known as stroke.
(a)
It contributes to the balance of physical–chemical properties of lipid membrane dynamics in cells; this includes the plasma membrane and the intracellular membranes .
(b)
It is a part of the bile acid synthesis; bile acids are important to absorb the lipids existing in food after ingestion.
(c)
It is also a part of the synthesis of vitamin D and hormones as important as estrogen in women and testosterone in men.
Different lipoproteins transport cholesterol to different destinations (see Sect. 3.1.2). Cholesterol associated to HDL (high-density lipoproteins) can be disposed to form bile acids, whereas the cholesterol associated to other lipoproteins such as LDL (low-density lipoproteins) cannot. This has been referred to as good (“HDL-associated”) and bad (“non-HDL-associated”) cholesterol. The distinction simplifies medical communication to lay audiences but is absolutely nonsense from the biochemical point of view. Cholesterol synthesis, distribution, and transformation can be considered a whole and interconnected process in the human body.
The most eloquent demonstration of how cholesterol is indispensable is the Smith–Lemli–Opitz syndrome. This is a rare disease characterized by failure to thrive; mental retardation; visual problems; physical defects in hands, feet, and/or internal organs; increased susceptibility to infection; and digestive problems, among others. Smith–Lemli–Opitz syndrome is a genetic disease caused by a defect in cholesterol synthesis, namely, deficiency of the enzyme 3β-hydroxysterol-Δ7-reductase, the final enzyme in the sterol synthetic pathway that converts 7-dehydrocholesterol (7DHC) to cholesterol (the complete pathway of cholesterol synthesis is described in Box 8.8). This results in low plasma cholesterol levels and elevated levels of cholesterol precursors, including 7DHC.
3.1.1 The Structure of Biological Membranes
The concept illustrated in Fig. 3.2 can be extended to the association of many lipid molecules. The entropic effect will cause the molecules to self-associate orderly, ultimately forming bilayers of lipids with a hydrophobic core (Fig. 3.7). If these bilayers are extensive enough for the overall structure to bend, then the bilayer curves allowing the sealing of the hydrophobic borders. All hydrophobic aliphatic chains become protected from water because the only areas in direct contact with the surrounding aqueous environment are the external and internal (“luminal”) surfaces formed by polar head groups of the lipids. This is depicted in Fig. 3.7.
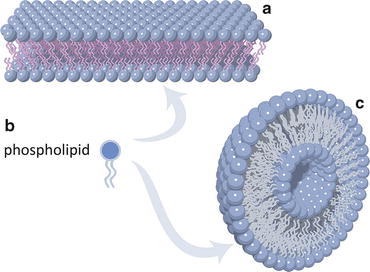
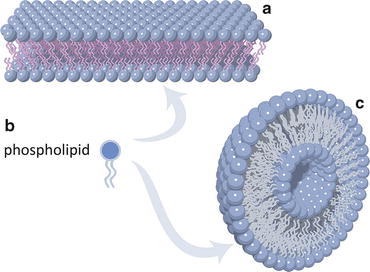
Fig. 3.7
Lipid molecules with a nearly cylindrical shape as a whole, such as phospholipids (b), tend to self-associate orderly due to the entropic effect (Fig. 3.2). Extensive self-association forms lipid bilayers (a) that ultimately bend and curve to self-seal into vesicles (c). Vesicles are the simplest prototype of cell membranes
Lipid vesicles form spontaneously and they are the simplest models of biological membranes . Amazingly, there is not a single cell known in nature that does not have their membranes formed with lipid bilayers to some extent. Diversity arises from the different kinds of lipids used and from their combination with other molecules, but the structural arrangement of the membrane itself is configured by lipid bilayers. Some cells (e.g., bacteria, fungi, and plants) may also have a cell wall in addition to the cellular membrane, which serves for structural and/or protection purposes.
In fact, lipid membranes are relatively malleable and fragile. Yet, such malleability and apparent fragility are extremely important characteristics from a dynamic point of view: membrane division and fusion, for instance, are favored as these processes do not imply covalent bonds to be broken or formed among lipids , and lipid bilayers are flexible. Membrane fusion is advantageous in many biological circumstances (Fig. 3.8). In biotechnology, mainly in pharma and cosmetics, lipid vesicles are valuable tools due to their properties (Box 3.2).
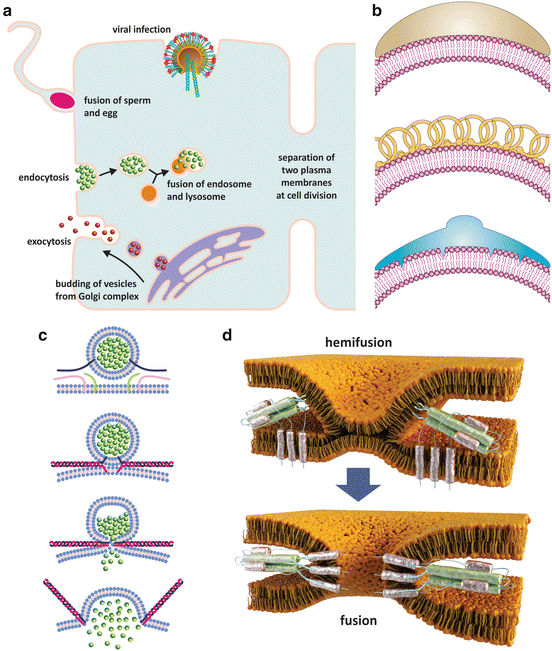
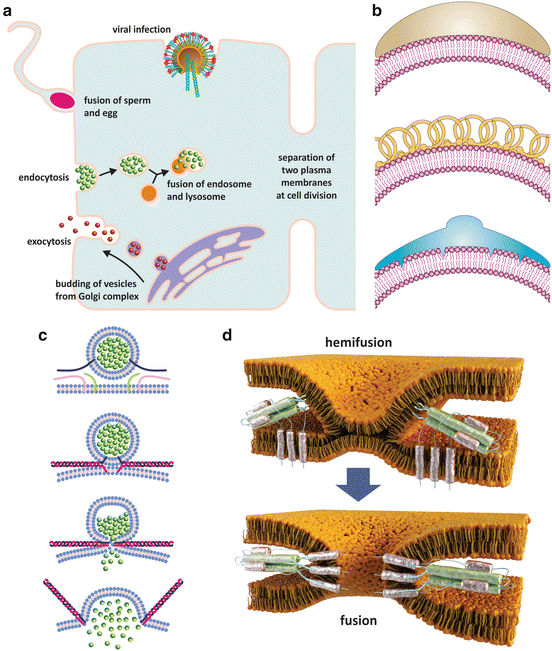
Fig. 3.8
Lipid membrane fusion occurs in nature associated with a plethora of cellular events (a). Some of these events such as endocytosis or budding of vesicles from the Golgi complex require inducing a curvature in membranes so that vesicles are formed. This induction is accomplished by the action of specialized proteins (b) that are curved and adhere to the surface of lipid bilayers (b, top), stabilize spontaneous curvatures (b, middle), or insert into one leaflet of the bilayer only thus forcing the membrane to bend (b, bottom) (reproduced with permission from Zimmerberg & Kazlov, Nature Rev Mol Cell Biol 7: 9–19, 2006). Membrane fusion implies that two different bilayers come together (c, d), which is due to the action of proteins that insert the two bilayers and promote conformational changes that lead to the contact of the lipid bilayers. First, a common bilayer is formed from the mix of the two membranes (hemifusion state), and then total fusion merges the two entities that were initially enclosed in their own membranes. Panel (c) shows the example of membrane fusion during neurotransmission, namely, a neurotransmitter being released at a synapse. The proteins responsible for fusion are named SNAREs (represented by green, pink, and blue filaments). Panel (d) shows the details of hemifusion and fusion of an envelope virus such as influenza virus, HIV , or dengue virus with the target cell. In this case viral proteins at the surface of the virus insert the membrane of the target cell and undergo conformational changes ending in fusion and consequent release of viral contents in the cytosol
Because lipid bilayers are the basic structure of cell membranes , several drugs directed to bacteria and fungi target the organization of lipid bilayers of these pathogens. Several antimicrobial peptides , for instance, are cationic so they bind and disrupt the lipid membranes of bacteria, which are highly anionic. Polyene antibiotics such as nystatin B and amphotericin B bind specifically to ergosterol disrupting the selective membrane permeability and ultimately causing the lysis of fungi (Fig. 3.9) because ergosterol only exists in the membrane of fungi.
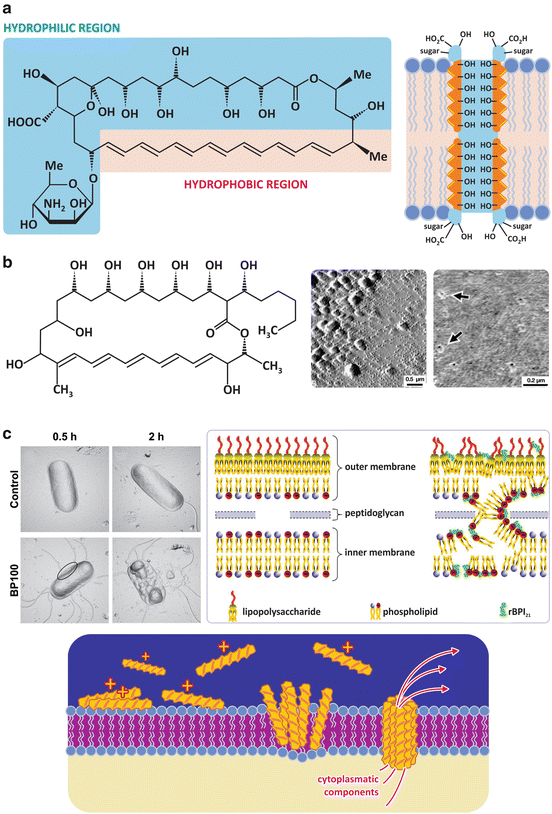
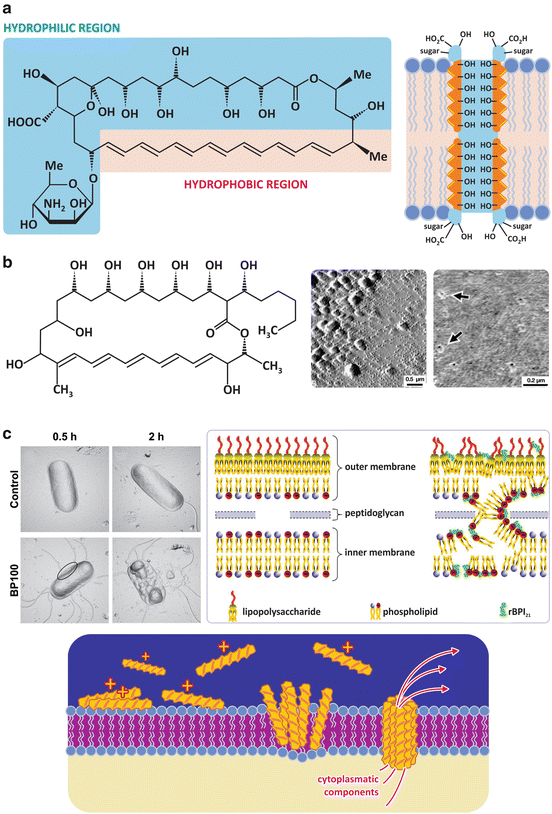
Fig. 3.9
Some drugs such as amphotericin B (a), a fungicide used in the treatment of infections with Candida sp. among others, or filipin (b), a fungicide also toxic to human cells and therefore not used in therapies, and cationic amphipathic peptides with antibacterial properties (c) target cell membranes . Amphotericin B binds to ergosterol forming ordered complexes in which the hydrophobic sides of the polyene rings face the lipids and the polar sides face each other forming a hydrophilic pore (a, right). Filipin is not so selective for ergosterol when compared to cholesterol. Amphotericin B, therefore, it is more toxic to human cells. Panel (b) (right) shows atomic force microscopy images of pores created by filipin in cholesterol-containing bilayers (arrows). Large structures in the surface of the lipid bilayer (b, center) are filipin aggregates. Panel (c) left also shows atomic force microscopy images but of an individual bacterium ( E. coli ). Exposure of the bacterium to the cationic amphipathic peptide BP100 caused the collapse of the bacterial membrane . The bacterial membrane is anionic, thus attracting electrostatically the peptides, which then aggregate on the lipid bilayer causing perturbation and increasing permeability. This perturbation may be caused by formation of pores or by unspecific destruction of the lipid organization (c, right, shows the action of rBPI21, a peptide derived from bactericidal/permeability-increasing protein potentially useful against meningitis). (Figures in panel (b) right are reprinted with permission from Santos et al., Biophys J. 75:1869–1873, 1998. Figures in panel (c) left are reprinted with permission from Alves et al., J. Biol. Chem. 285:27536–27544, 2010. Figures in panel (c, right) are reprinted with permission from Domingues et al., PLoS ONE, 4:12, e8385, 2009
While lipid vesicles depicted in Fig. 3.7 are formed only by lipids (a single pure lipid or a mixture of lipids), biological membranes are often composed of lipids, proteins , and saccharides . How these components organize in the membrane has been the subject of intensive scientific research over the years (Fig. 3.10). Nowadays, a biological membrane of a human cell is regarded as a lipid bilayer having on heterogeneous distribution of lipids both in each layer and among layers. This heterogeneity leads to the formation of specific domains of lipids having defined functions. Rigid platforms, for instance, may serve to anchor proteins on the membrane (Fig. 3.11). The cell membrane is directly connected to the cytoskeleton through an array of proteins, the so-called cytoskeleton anchors. The outer surface of cell membranes may have lipids and proteins that are glycosylated (i.e., covalently attached to saccharides) contributing to a rich chemical diversity on the surface of cells (Fig. 3.10).
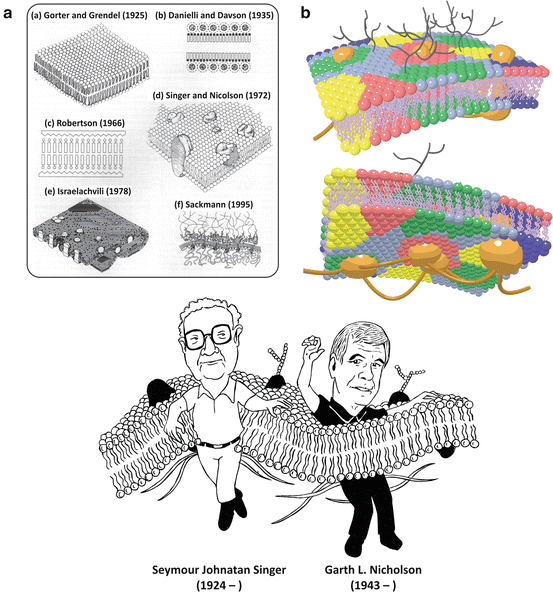
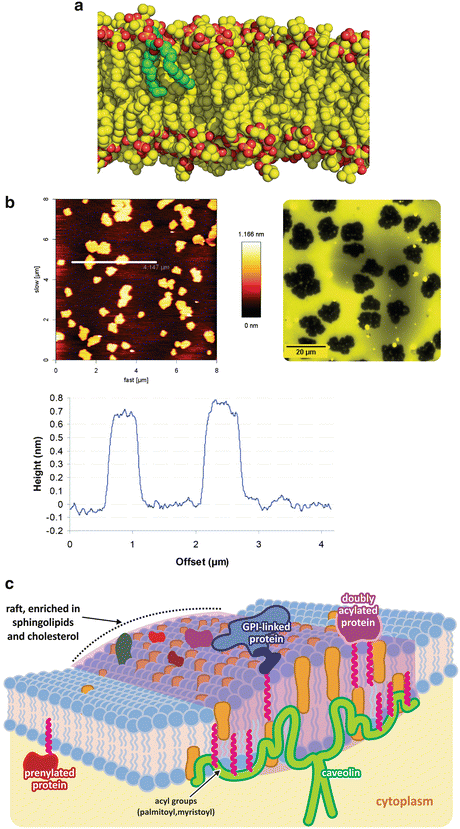
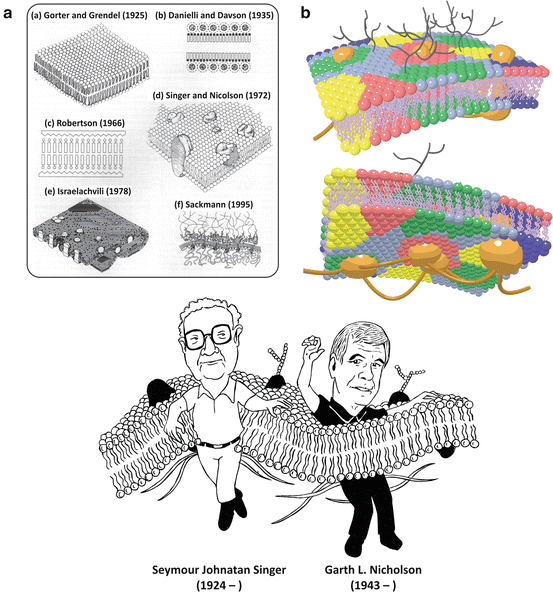
Fig. 3.10
(a) Historical evolution of the concept of cell membranes (according to Ole Moritsen; reprinted with permission from Biol. Skr. Vid. Selsk. 49:7–12, 1998). The lipid bilayer was first identified, followed by the discovery that proteins interact with lipids . The concept that there are integral proteins embedded in the lipid bilayer forming a dynamic structure came with the fluid mosaic model by Singer and Nicolson in 1972, which is still accepted as the basic framework of membrane structure. Nevertheless, from then on, the organization of cell membranes has been continuously unraveled. Lipids are now known to self-associate in lateral domains of different composition, and some membrane proteins are bound (“anchored”) to the cytoskeleton (A(e) and A(f)). The modern view on cell membranes is schematized in (b), in which lipid colors represent heterogeneous lipid compositions. In the outer surface of cell membranes (b, top), glycosylated (i.e., saccharide-containing, black) lipids and proteins are present with different functionalities (see Sect. 3.2). Cytoskeleton and cytoskeleton-binding proteins are represented in brown
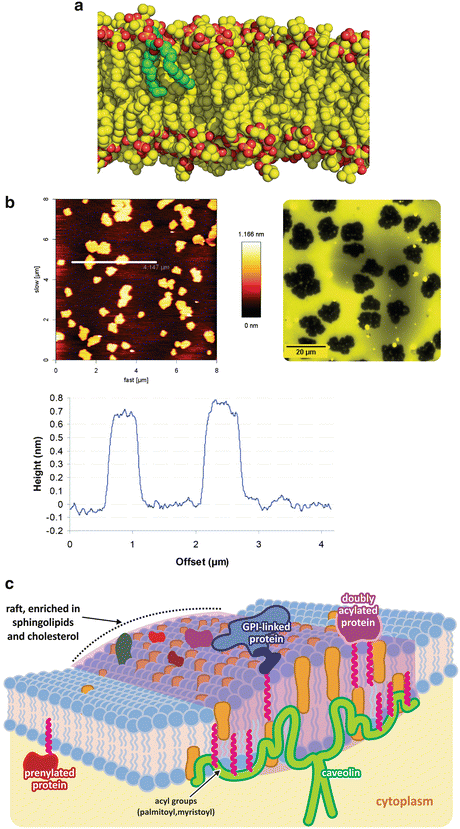
Fig. 3.11
Heterogeneity in lipid membranes . Although phospholipids are frequently depicted in an oversimplified form with ordered stretched aliphatic chains, as in Fig. 3.2, in reality most molecules in lipid bilayers in physiological conditions in cells have very flexible and dynamic acyl chains. Panel (a) shows molecular dynamics simulation of lipids with one single lipid in green to highlight the bent conformation (courtesy of Dr. Claudio Soares, ITQB-UNL, Portugal). Lipids with longer and saturated chains adopt stiffer and linear conformations as they interact more tightly with each other. Mixing stiff and fluid lipids results in partial segregation of the lipids. The atomic force microscopy image of a mixture of a fluid unsaturated lipid (palmitoyl-oleyl phosphatidylcholine, POPC—50 % molar) with a saturated rigid lipid (dipalmitoyl-phosphatidyl choline, DPPC) is shown in panel (b, left); segregated areas are clear, and the height profile along the line seen in the top image (bottom graph) shows that the segregated areas are higher, therefore corresponding to more rigid areas of the membrane . Epifluorescence images of lipid bilayers having one of the lipid tagged with a fluorescent dye confirm segregation of both lipids (b, right). Figure reprinted with permission from Franquelim et al., J. Am. Chem. Soc. 130:6215–6223, 2008; and Franquelim et al., Biochim. Biophys. Acta. 1828:1777–1785, 2013. These more rigid areas of the membrane are more adapted to anchor proteins and membrane receptors (c). Sphingolipids and cholesterol , for instance, further enhance these characteristics. Proteins covalently attached to lipids are typically found in these rigid platforms
The lipid composition of cell membranes varies a lot from species to species, from organelle to organelle in the same cell, and from the inner leaflet to the outer leaflet in the same membrane (Fig. 3.12). This variety of compositions grants the necessary diversity to membranes so that they are specific for certain functions, in spite of the common feature of all membranes of all cells: in the end they are all constructions based on lipid bilayers.
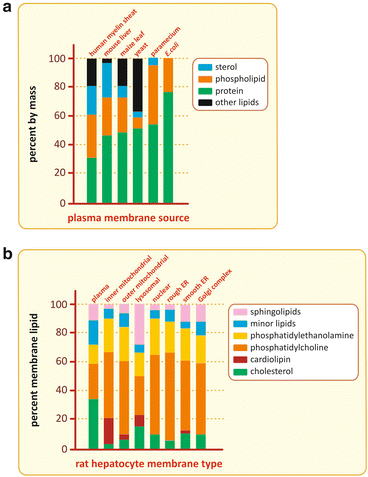
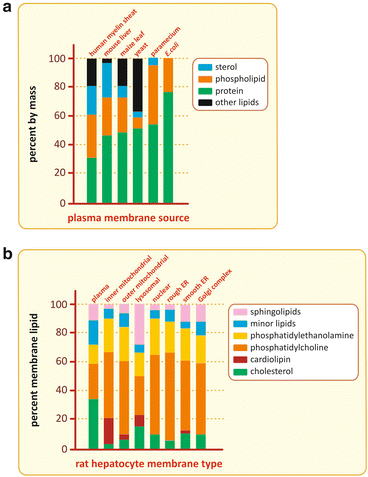
Fig. 3.12
Although all membranes in cells in nature are constructions based on lipid bilayers, the membrane composition varies a lot among species (top), among organelles of the same cell (bottom), and even between the two leaflets of the same membrane (not shown)
Because lipid bilayers are hydrophobic barriers, hydrophilic molecules such as glucose cannot freely transverse them, but channels and transporters in their membranes overcome the limitation. Channels and transporters are proteins specific for certain molecules or ions that facilitate or enable the translocation of such molecules or ions across the membranes. This subject will be revisited in Sect. 5.3.1, after careful consideration of protein structure.
Box 3.2: Lipid Vesicles in Pharma and Cosmetics
For many decades, lipids were considered relatively inert biologically, with functions of storage of energy in the adipose tissue or constitution of a matrix for cell membranes . Thus, in general, there was little interest in research to discover the properties, structures, biosynthetic pathways, biological utilization, and other functions of lipids. In the present, the situation is completely opposite. Lipids are regarded as important biological molecules that in addition to being energy stores and membrane components, participate in the regulation of many biochemical processes in cells and endocrine physiological regulation in the human body. Moreover, lipids are now important tools in pharma and cosmetic industries because they can be used in formulations that distribute and deliver drugs or other biologically active molecules in the human body. In most of these formulations, the lipids self-assemble in bilayers that form extensive vesicular systems, liposomes (see figure), able to encapsulate molecules having desirable functions. Liposomes are very versatile systems as hydrophobic molecules may be accumulated in the lipid areas, and hydrophilic molecules may remain solubilized in the aqueous spaces inside vesicles.
In cosmetic applications, the liposomes may be part of formulations to be applied topically in skin. The lipids help in the diffusion of the formulation through the outer layers of skin. Moreover, the simple fact that lipids and water are forming an emulsion will help the formulation to hydrate the desired areas of the skin.
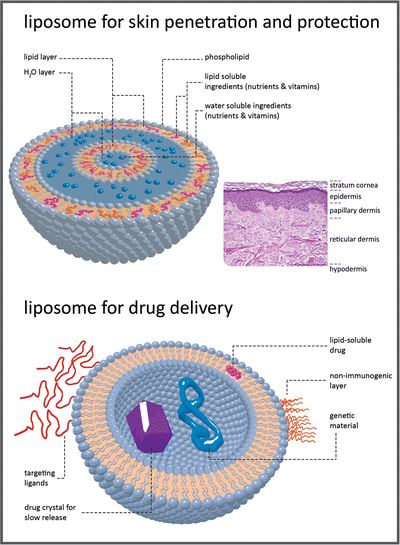
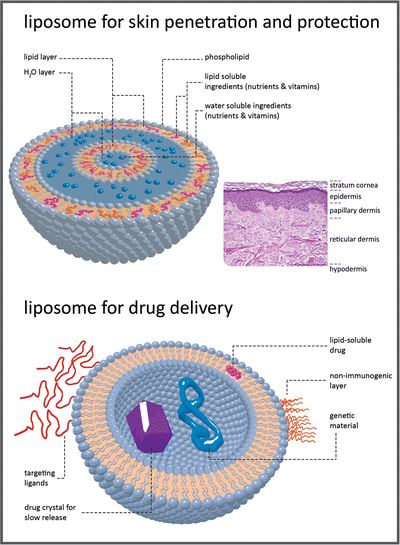
Liposomal encapsulation can substantially improve the action of a drug, such as the decreased toxicity observed with amphotericin B (see Fig. 3.9). Conventional amphotericin B has been generally considered the drug of choice for many types of systemic fungal infections. These infections are a major threat to those whose immune systems are compromised, such as patients undergoing chemotherapy for cancer, bone marrow transplant recipients and AIDS patients. However, amphotericin B is very toxic, thus limiting its utility. For these patients, who have a high rate of morbidity and mortality, there is a dosage form distinct from conventional amphotericin B, which consists of amphotericin B complexed with two phospholipids in approximately a 1:1 drug-to-lipid molar ratio: l-α-dimyristoylphosphatidylcholine (DMPC) and l-α-dimyristoylphosphatidylglycerol (DMPG), present in a 7:3 molar ratio. Doxorubicin is another example. Liposomal doxorubicin is designed to target to tumor cells and spare healthy tissue, maintaining efficacy while reducing toxicity. Conventional doxorubicin, drug commonly used to treat cancer, is limited by its potential for causing a variety of severe side effects, particularly irreversible heart damage.
Researchers are developing innovative liposomes with refined drug delivery properties to be part of future medicines. Some have their surface modified with proteins and other selected polymers to target selected cells. Synthetic phospholipids are suitable for specific applications in liposome targeting and gene therapy. Gene therapy is based on the efficient delivery of genes to their intended targets. Researchers have successfully put DNA into liposomes and have achieved fusion of these liposomes to cells. Scientists have also succeeded in protecting these liposomes from degradation and are able to modulate their circulation time.
3.1.2 The Structure of Lipoproteins
Lipoproteins are organized assemblies of lipids and proteins covering a wide range of sizes and densities (Fig. 3.13). These assemblies have a lipid ic core formed mainly by triacylglycerols and cholesterol esters, covered by a monolayer of phospholipids . The global arrangement is largely determined by the entropic effect as the monolayer of phospholipids minimizes the contact between apolar components and water molecules in blood (Box 3.3). Lipoproteins transport lipids between different tissues. There are proteins inserted in the lipid layer, exposed at the surface of the lipoprotein, as shown in Fig. 3.14.
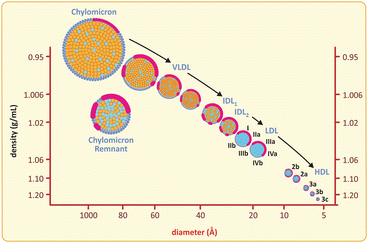
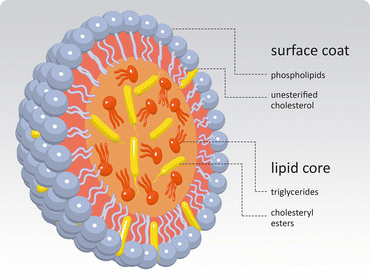
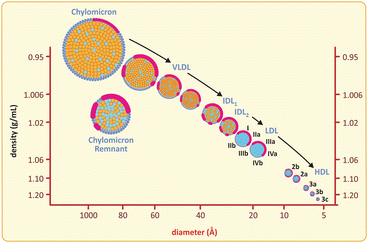
Fig. 3.13
Lipoproteins are grouped according to their densities and sizes, although the most common nomenclature refers to density ( HDL high-density lipoprotein, LDL low-density lipoprotein, VLDL very-low-density lipoprotein). Chylomicra are the structures formed with dietary lipids in the enterocyte (intestines) and released in plasma
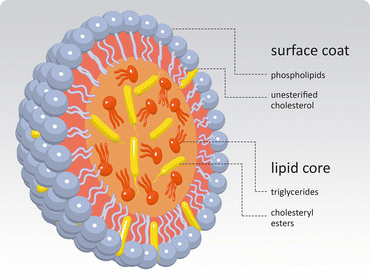
Fig. 3.14
Global generic structure shared by all classes of lipoproteins. A monolayer of phospholipids and cholesterol covers a lipid droplet of triacylglycerols and cholesterol esters. There are proteins at the surface (not shown), the apolipoproteins , which are specific of each class of lipoproteins and serve for cell recognition, i.e., interaction with specific receptors in cells
Box 3.3: Lipoproteins: The Burden of Lipid Transport
Lipids have extremely low solubility in aqueous media. Therefore, as a consequence of the entropic effect (see Sect. 3.1), when placed in aqueous medium, they tend to self-associate. Most phospholipids , having a hydrophilic “head” and two hydrophobic acyl chains, tend to pack side by side and form bilayers. Cholesterol is not prone to form very organized supramolecular assemblies itself but is able to insert in the lipid bilayers and contribute to its stability. Triacylglycerols (“triglycerides”) and esters of cholesterol (cholesteryl esters; see figure) do not have the amphipathic properties and structural requirements to form lipid bilayers. Instead, triacylglycerols and cholesteryl esters amalgamate in an aggregate having no polar surface. These aggregates tend to be spherical, the geometry that minimizes the surface area exposed to the solvent. The lipid aggregates tend to grow until free lipid is nearly absent unless a phospholipid monolayer covers the surface of these aggregates, forming an entropically favorable interface. The phospholipid monolayers stabilize the lipid aggregates and an emulsion is formed. Emulsion means the lipids are heterogeneously distributed in microscopic scale because the lipids are clustered in aggregates but homogeneously distributed in macroscopic scale since the aggregates are evenly disseminated in the solvent.
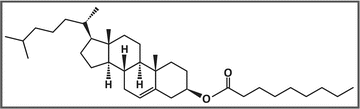
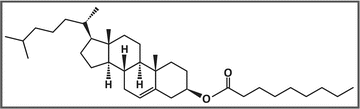
Example of a cholesteryl ester: cholesteryl nonanoate. The molecule is composed of a cholesterol moiety and a fatty acid moiety (nonanoic acid in this case)
In human body, very large lipid aggregates are found in the cells of the adipose tissue (adipocytes), occupying almost all cytoplasmic space. It is a storage place. Smaller aggregates are found emulsified in blood, in association with specific proteins . These smaller aggregates are dragged by blood and serve as lipid transporters. In both cases, the aggregates are covered by monolayers of phospholipids having the polar groups exposed to aqueous environment and the acyl “tails” in contact with the lipids . The ensemble formed by the emulsified lipid aggregate covered with a phospholipid monolayer and associated to specific proteins is named lipoprotein (see Fig. 3.14 in the main text). The proteins themselves are named apolipoproteins . The lipoprotein as a whole is the lipid carrier entity; apolipoproteins bind to specific receptors so that lipids are delivered to target cells only.
Lipoproteins vary among them in the proportion of triglycerides/cholesteryl esters/protein , which directly interferes in their compactness and density. Early studies on the properties of lipoproteins achieved separation of several classes of lipoproteins based on their different densities, so the density-based nomenclature was naturally adopted, from high-density lipoproteins (HDL ) to very-low-density lipoproteins (VLDL) and chylomicra (see below table). There is a concomitant change in volume but it is not given importance regarding lipoprotein classification. It is also worth highlighting that apolipoproteins are also divided in classes (A, B, C, etc.) and HDLs are the only lipoproteins not bearing apolipoproteins B, which is a distinctive feature.
Properties of plasma lipoproteins
Plasma lipoproteins | Density (g ml−1) | Diameter (nm) | Apolipoprotein | Physiological role |
---|---|---|---|---|
Chylomicron | <0.95 | 75–1200 | B48, C, E | Dietary fat transport |
Very-low-density lipoprotein | 0.95–1.006 | 30–80 | B100, C, E | Endogenous fat transport |
Intermediate-density lipoprotein | 1.006–1.019 | 15–35 | B100, E | LDL precursor |
Low-density lipoprotein | 1.019–1.063 | 18–25 | B100 | Cholesterol transport |
High-density lipoprotein | 1.063–1.21 | 7.5–20 | A | Reverse cholesterol transport |
During digestion, the lipids are partially degraded and emulsified in the intestinal lumen by bile acids, molecules similar to cholesterol but having several polar groups. Lipids and other nutrients are taken up by the intestinal cells, enterocytes (see figure). Chylomicra are formed in these cells and released in the blood. Eighty to ninety percent of the lipids in chylomicra are triglycerides, which account for their low density. The remaining cargo is free cholesterol (1–3 %), cholesteryl esters (3–6 %), and phospholipids (7–9 %). Chylomicra circulate in the blood, where degradation of their triacylglycerides into free fatty acid s occurs. Part of these fatty acids is delivered to adipose tissue and peripheral tissues. The chylomicra remnants bind to liver cells having specific receptors. The excess of nutrient incorporated after digestion is converted to lipids in the liver, where they form VLDLs in a process similar to the assembly of chylomicra in the intestine. VLDLs are released from the liver cells. In the bloodstream, they are depleted of free fatty acids from triacylglycerols, resulting in the intermediary density lipoproteins (IDLs) and low-density lipoproteins (LDLs), which transfer lipids to peripheral tissues bearing LDL receptors. Liver cells having LDL receptors also bind LDL. In contrast, HDLs transport cholesterol from peripheral tissues to the liver, where there are cells having specific receptors for HDL . The cholesterol is then used to synthesize bile acids. HDLs are the only lipoproteins that dispose of cholesterol. This characteristic renders the name “good cholesterol” to HDL-associated cholesterol in public health campaigns for lay audiences. This name makes no sense on biochemical grounds but helps to spread the message that in cardiovascular risk evaluation, it is important to differentiate between cholesterol that is being removed and cholesterol that is being incorporated. Interestingly from the biochemical point of view, cholesterol to be disposed is associated to lipoproteins having no apolipoprotein B and cholesterol to be incorporated is associated to apolipoprotein B-containing lipoproteins.
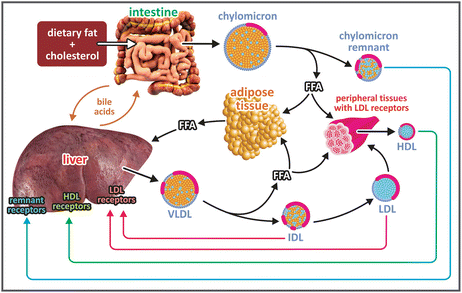
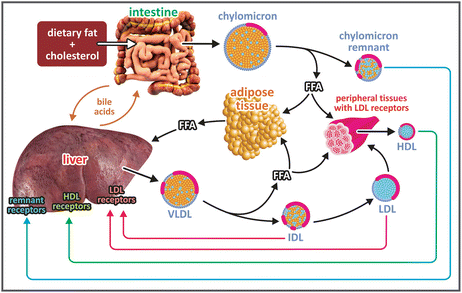
Origin and fates of plasma lipoproteins
LDLs are degraded inside the cells after being taken up by endocytosis. The LDL receptor is segregated in the endocytic vesicle, which then divides in two: one empty vesicle having the receptors in the membrane returns to the surface of the cell, and the other vesicle has the proteins and lipids of the lipoproteins and fuses with the lysosome. LDL-derived cholesterol may then be used either in cell membranes , or as a precursor to synthesize steroid hormones or bile acids, or simply be stored as cholesteryl esters. The exact destination of cholesterol in the cell depends on the type of cell and its metabolic state. Dietary cholesterol suppresses the synthesis of cholesterol by the body, and high free cholesterol levels inhibit the synthesis of LDL receptors. Cellular uptake is thus inhibited in the presence of excess cholesterol, and the level of LDL in the blood increases. Moreover, the LDLs take more time to be uptaken and circulate in blood for longer periods. This increases the chances of having the LDL exposed to oxidative agents such as NO, hydrogen peroxide, or the superoxide ion. Oxidized LDLs are then removed from circulation by macrophages, but the macrophages get their properties severely altered, becoming the so-called foam cells. Foam cells accumulate in the walls of endothelia, releasing growth factors and cytokines that stimulate the migration of smooth muscle cells that proliferate in the site of accumulation of foam cells and form collagen matrices. This consists of the deposition of atherosclerotic plates, which poses a severe cardiovascular risk.
Interestingly, the proteins responsible for triacylglycerol conversion into fatty acid s in the heart (enzymes heart lipoprotein lipases ) have a much higher affinity for triacylglycerols than the corresponding proteins in the adipose tissue. The affinity parameter, 1/ K M , which will be addressed in Sect. 4.2.1 is about tenfold higher in the heart. During starvation the levels of plasma triacylglycerol drop, but delivery of fatty acids from triacylglycerols is kept in the heart even when suppressed to the adipose tissue.
Different classes of lipoproteins differ in density (due to differences in the relative amounts of proteins , phospholipids , cholesterol , triacylglycerols, and cholesterol esters in their composition), size, and specific proteins associated (see Table in Box 3.3). Nevertheless, these classes are named after the differences in density only, which relates to the most practical property that can be used for their separation in different fractions (see Fig. 3.13). Lipoproteins formed in the intestine with dietary lipids are known as chylomicra, and the remaining classes range from very-low-density lipoproteins (VLDLs) to high-density lipoproteins (HDLs). Intermediate-density lipoproteins (IDLs) and low-density lipoproteins (LDLs) are in between.
The different classes of lipoproteins have different functions and different target tissues for their action (see Box 3.3). Target recognition depends on the specificity of the proteins present on the lipoprotein surface (referred to as apolipoproteins to highlight that only the proteic part is being addressed) for well-defined receptors.
Other lipid ic supramolecular assemblies, such as lipid droplets in the liver and lipid depots in adipose tissue, exist. Lipid depots may occupy most of the volume of an adipocyte, the adipose tissue cells (see Fig. 3.3), confining the cytoplasm to a very small fraction of the cell. These lipid depots are also covered by phospholipids that are stabilized as a consequence of the entropic effect.
3.2 Saccharides and Their Polymers and Derivatives
Saccharides, different from lipids are extremely polar, therefore, hydrophilic, molecules. They are linear aldehydes or ketones with hydroxyl groups bound to the carbons that do not form the carbonyls (C=O). Many of these molecules have only C, H, and O in their composition and fit the formula (CH2O) n . This spurious characteristic consecrated the designation “carbon hydrate”, which is still widely used to identify saccharides despite its total inadequacy in chemical terms: not all saccharides obey to (CH2O) n and this does not reflect an hydration of carbon, only a specific molar proportion between C, H, and O atoms. Referring to saccharides as “sugars” is equally inadequate and misleading. “Sugar” is related to a property, sweetness, which not all saccharides possess and extends to molecules other than saccharides, such as peptide sweeteners (Box 3.4). Saccharides or oses are therefore the preferred nomenclatures for biochemists although “carbon hydrates”, or carbohydrates, in short, and “sugars” are commonly used in closely related areas such as nutrition, for instance.
Box 3.4: Sweeteners and Sugar Substitutes
The problem of popularization of high caloric diets stimulated the search for sugar substitutes. Sucrose, the most commonly used sugar in cooking, is a natural sweet molecule from which a certain amount of energy can be used by the human body after metabolization. However, there are molecules known as high-intensity sweeteners that have manyfold the sweetening power of sucrose. Saccharin, for instance, is approximately 300-fold sweeter than sucrose when equal quantities are compared. Aspartame and acesulfame-K are approximately 200-fold sweeter than sucrose. For sucralose the ratio raises to an impressive 600-fold. A specific chemical modification in aspartame, advantame, grants an impressive 20,000-fold increase in sweetness relative to sucrose. Therefore, much less mass of sweetener is needed to achieve the sweet taste of a food or beverage. Even though the “caloric content” of a unit mass of the molecule may be equivalent to sucrose in some cases, the total amount used is several orders of magnitude less and the total calories in the diet drops drastically.
The chemical structure of sucrose and most artificial sweeteners are very different. Sucrose is a disaccharide composed of the residues of the monosaccharides glucose and fructose . Sucralose is prepared from sucrose via the substitution of three hydroxyl groups for three chlorides. Saccharin and acesulfame-K have much different structures. Aspartame is the methyl ester of the dipeptide l-aspartyl-l-phenylalanine.
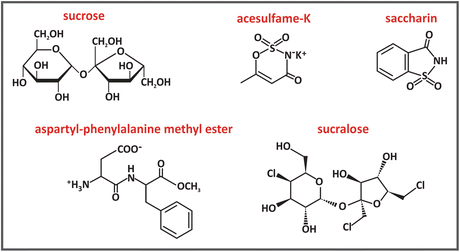
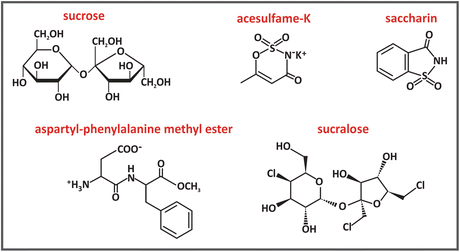
The molecular structure of sweeteners must be such that they bind to a specific receptor molecule at the surface of the tongue. The receptor is coupled to a G protein (see Sect. 5.4), which dissociates when the sweetener binds to the receptor. This dissociation leads to the activation of an enzyme that triggers a sequence of events resulting in signals that are carried to and interpreted by the brain. The sweetness perception depends on fine details of the interaction between receptor and sweetener. The importance of molecular shape to sweetness is illustrated by the case of aspartame, as its stereo isomer, l-aspartyl-d-phenylalanine methyl ester, has a bitter, not a sweet, taste.
There has been a long and continuous controversy about the impact of artificial sweeteners on health, which has driven a lot of research on the possible toxicity of their metabolic products. Saccharin has been very controversial and banned in some countries. In the body, aspartame is broken down into/absorbed as products that include aspartate, phenylalanine, and methanol. Phenylalanine is toxic to individuals with phenylketonuria, a genetic disease wherein individuals cannot process phenylalanine. Products containing aspartame must therefore be labeled for phenylalanine. Aspartame’s breakdown products—phenylalanine and aspartate, as well as methanol and its breakdown products formaldehyde and formate—are a matter of debate. Regardless of the controversies and limitations in their use, artificial sweeteners have an important role in the improvement of the quality of life of diabetics, who are limited in the consumption of sucrose and other saccharides .
Saccharides are the most abundant biomolecules and owe this ubiquity to their reactivity and structural plasticity, which enable a great variety of functionalities, including energetic storage, cell communication, and cell protection against mechanical aggressions and dehydration. In order to understand such structural plasticity and the functionalities arising from it, one has to start with the basic chemistry and reactivity of saccharides . Although this is a wide and complex area in the realm of biochemistry, we will devote ourselves to the understanding of the most important saccharides in human biochemistry only. One will stick to the basics of this fascinating world for the sake of clarity and focus on processes that are foundational for other medical disciplines such as histology and physiology.
The simplest conceivable saccharides have three-carbon chains, i.e., they are trioses (“tri” for three carbons, “ose” for saccharide). Depending on the position of the carbonyl group, C=O, which may be terminal (aldehyde) or not (ketone), the saccharide is an aldose or a ketose. In the specific case of trioses, only glyceraldehyde and dihydroxyketone are possible (Fig. 3.15). But even in these cases, two kinds of common chemical reactions in nature are possible: esterification and reduction (Fig. 3.16).
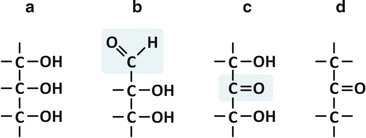
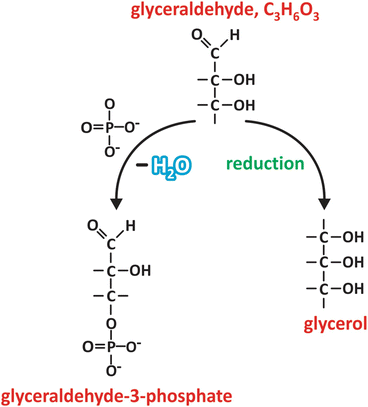
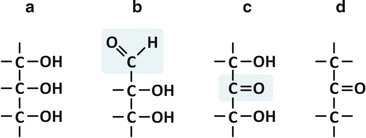
Fig. 3.15
Glycerol [(a); also in Fig. 3.4d] is related to an aldehyde, glyceraldehyde (b), which has a ketone isomer, dihydroxyketone (c), which in turn is related to a ketone (d), dimethyl ketone. The aldehyde and ketone groups of glyceraldehyde and dihydroxyketone are highlighted in the chemical structures (b) and (c), respectively, by a shadowed box
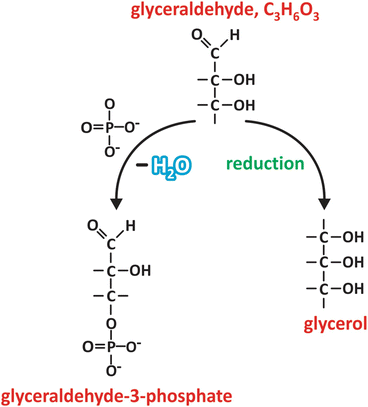
Fig. 3.16
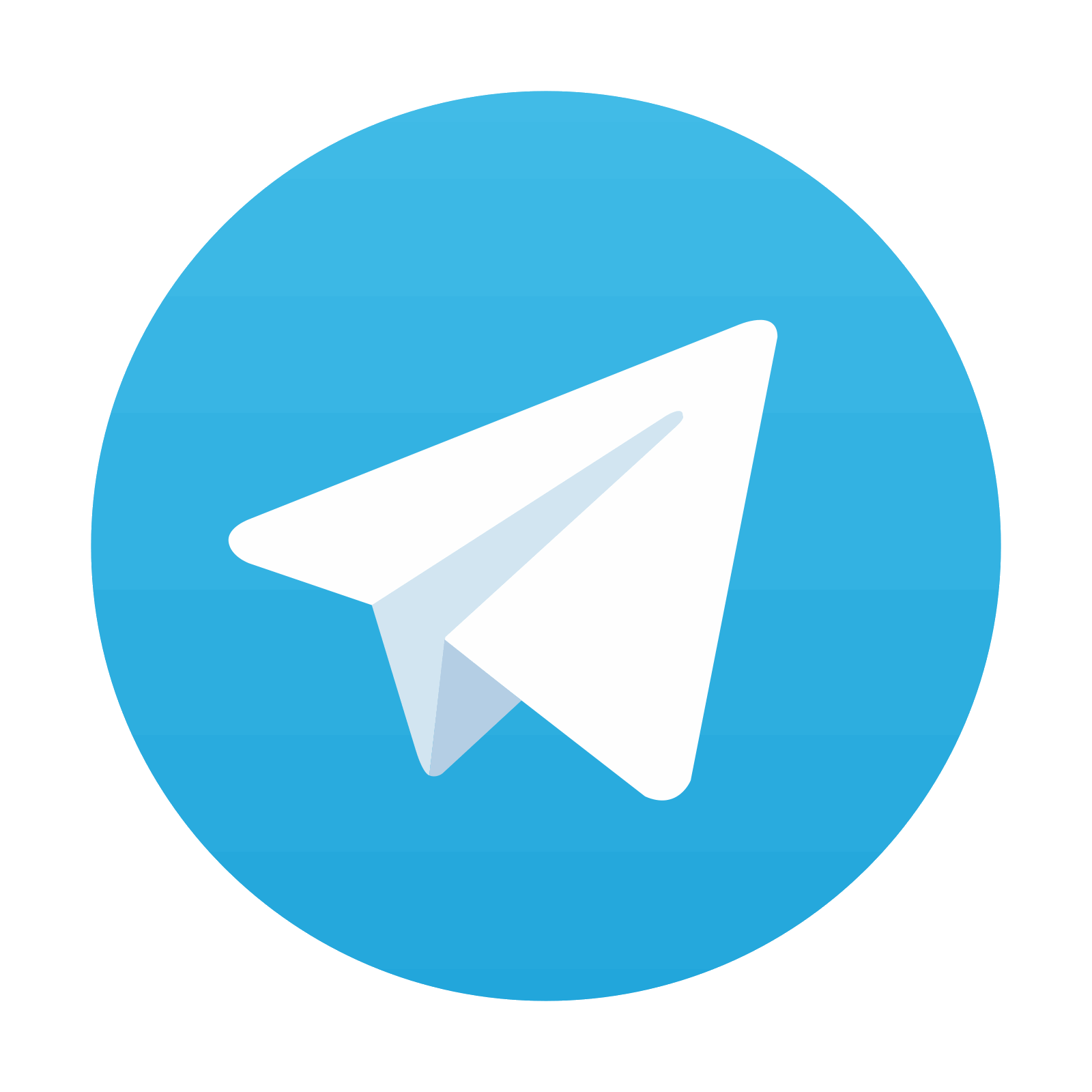
Glyceraldehyde may be reduced to glycerol upon chemical reduction of the C=O group in carbon 1 (carbons are numbered starting with the one from the carbonyl group, similarly to fatty acid s , in which carbons are numbered starting in the carboxyl group). Phosphoric acid (HPO4 2−) may react with carbon 3, for instance, to form an ester, glyceraldehyde-3-phosphate. These are examples of very frequent reactions involving saccharides
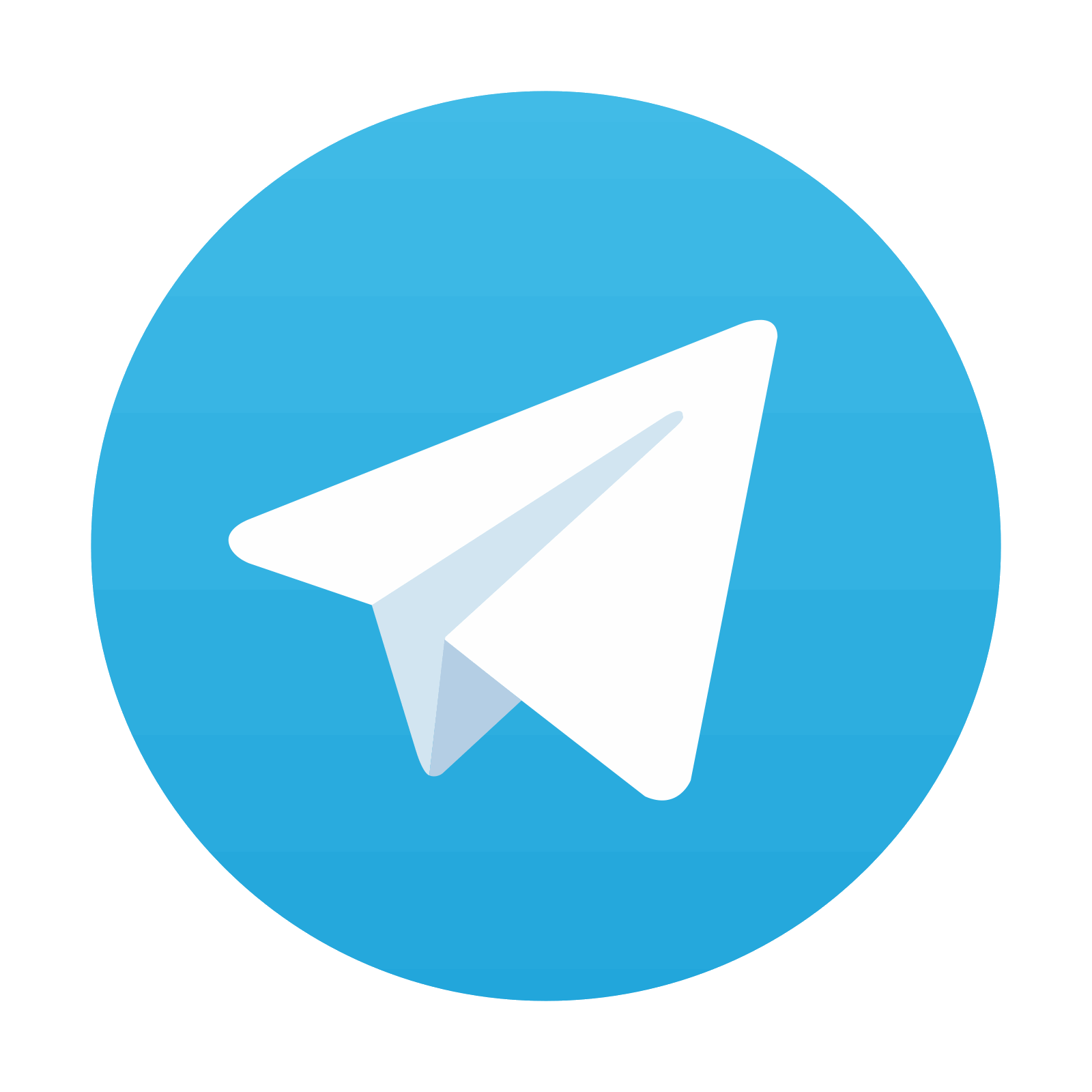
Stay updated, free articles. Join our Telegram channel
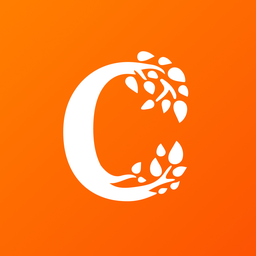
Full access? Get Clinical Tree
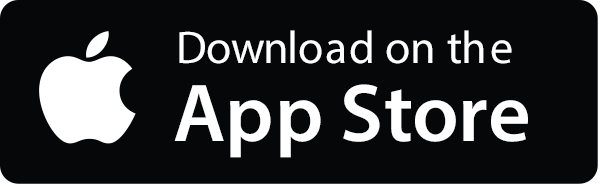
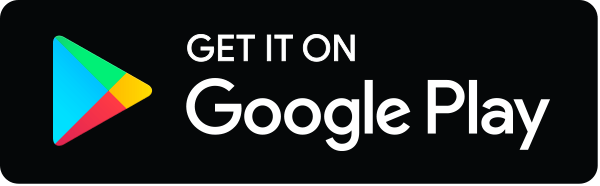
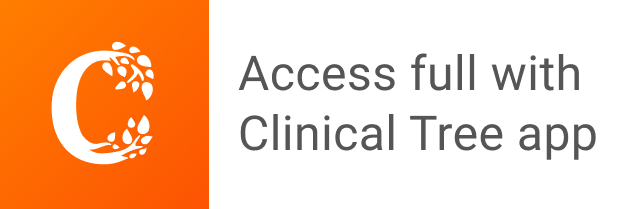