16 Tom P. Fleming and Congshan Sun During the first 5–7 days of life, the early embryo undertakes a complex, challenging developmental programme, set within a tight deadline and comprising a daunting ‘tick list’ of activities which have been well studied mainly across animal models, with more limited data on the human. The embryo programme of activities can be briefly summarized as: The embryo is involved in more than this busy series of classical, inward‐looking developmental transitions. Recent research indicates a second more outward‐looking programme running in parallel where the embryo interfaces with its environment. This second series of events confers plasticity of the emerging phenotype to promote an optimal growth trajectory and metabolic status best suited to the actual conditions the embryo is experiencing. We believe external conditions such as maternal nutrition, physiology, and health status can be ‘sensed’ by early embryos resulting in adaptations that support survival and competitiveness of the offspring. These in vivo interactions and modifications can also occur in vitro, and so are relevant within the clinical setting of assisted reproductive technology (ART) (Figure 16.1). Such sensing and response mechanisms can be considered as embryos making ‘decisions’ on their future or may reflect perturbations in the inherent programme caused by external factors (Fleming et al. 2015) and stress conditions (Puscheck et al. 2015). This second, extrinsic, programme is the subject of this chapter. Embryo environmental programming has profound consequences with a legacy that persists into late adult life and may associate with increased disease risk, notably cardiometabolic and behavioural. It is clear that the combination of inward‐ and outward‐looking developmental programmes conducted by the early embryo represents a critical phase in the life course and that it is essential to explore further. Figure 16.1 Summary of periconceptional developmental programming mediated through maternal and in vitro environmental cues that are sensed by embryos resulting in induction of programming and a series of responses or adaptations in embryonic and extra‐embryonic cell lineages. Collectively, through cellular, physiological and epigenetic mechanisms, the developmental programme is altered, leading to fetal and postnatal changes in growth and phenotype and ultimately, the origin of health and disease in offspring. Maternal physiology and dietary nutrients, both over‐ and undernutrition, affect follicular and maturing oocytes and early embryos in ways that can permanently affect developmental potential (Sinclair and Watkins 2013). Maternal obesity is well recognized to reduce fertility, increase early pregnancy loss, and promote the risk of congenital abnormalities (Grindler and Moley 2013). Maternal circulating lipids can influence the accumulation of lipid metabolites in follicular fluid and the oocyte. For example, it has been shown that follicular fluid triglyceride concentrations are positively correlated with maternal body mass index (Robker et al. 2009). There is also substantial evidence from small and large animal models that fatty maternal diets lead to increased follicular and oocyte lipid levels with detrimental consequences for oocyte and embryo potential (Dunning et al. 2014). Thus, maternal high fat diet in mice may increase follicular and oocyte lipid content leading to impaired fertilization and poorer embryo quality (Wakefield et al. 2008; Wu et al. 2010) and later fetal abnormalities (Luzzo et al. 2012). Data consistent with these conclusions has been obtained by Leary et al (2015), who related maternal body mass index to the triglyceride content of single blastocysts conceived via in vitro fertilization (IVF). The major cellular defect in oocyte and embryo potential mediated through high fat diet and obesity relates to mitochondrial function, which becomes compromised and associated with increased oxidative and endoplasmic reticulum stress (Igosheva et al. 2010; Wu et al. 2012). These adverse effects on mitochondria following an over‐rich diet also include impaired morphology and biogenesis (Igosheva et al. 2010; Luzzo et al. 2012). The longer‐term outcomes of maternal obesity and high fat nutrition across animal models have demonstrated that adverse periconceptional programming leads to cardiovascular and metabolic disease in the offspring (Samuelsson et al. 2008; Picone et al. 2011; Torrens et al. 2012) as well as compromising ovarian morphology and function (Cheong et al. 2014) and perpetuating the condition across generations. With others, we have focused on the mechanistic basis of maternal undernutrition effects on embryo potential; it is useful to break these down into long‐term consequences, early causes, and potential epigenetic regulation. Maternal undernutrition during the periconceptional period has been extensively studied using the mouse isocaloric low protein diet (LPD) model, including maternal protein restriction exclusively during the period of oocyte maturation (Egg‐LPD) or preimplantation development (Emb‐LPD) and with normal nutrition at all other times and postnatally (Watkins et al. 2008a; Watkins et al. 2008b). As has been discussed for maternal overnutrition, these dietary treatments lead to increased risk of cardiometabolic disease in the adult offspring, notably hypertension, endothelial vascular impairment, nephron deficit, and increased angiotensin‐converting enzyme (ACE) activity, in a gender‐specific manner (Watkins et al. 2008a; Watkins et al. 2008b; Watkins et al. 2010; Watkins et al. 2011). The Emb‐LPD offspring, especially females, also exhibit impaired energy metabolism with excess adiposity and a gene expression profile that indicates a storage, anabolic metabolism (Watkins et al. 2011). A similar cardiometabolic phenotype occurs in Emb‐LPD rat offspring (Kwong et al. 2000). Moreover, Emb‐LPD and Egg‐LPD adult offspring display a disturbed behavioural phenotype that influences the level of locomotory activity (Watkins et al. 2008a; Watkins et al. 2008b). These diverse adult abnormalities appear to derive from an altered growth trajectory during gestation since the perinatal weight of offspring is positively associated with adult weight and cardiovascular and behavioural conditions (Watkins et al. 2008a). The sensitivity of periconceptional maternal undernutrition to adult health has also been explored in sheep and similar adverse cardiovascular, metabolic, and behavioural outcomes have been identified (Gardner et al. 2004; Hernandez et al. 2009; Todd et al. 2009; Torrens et al. 2009). Most critically, in limited human maternal nutritional datasets, there is further evidence that periconceptional or early gestational exposure to famine leads to a cardiometabolic and neurological phenotype in adult offspring, which is more severe than exposure during later stages of pregnancy (Roseboom et al. 2011). The apparent consistency in embryo vulnerability to poor maternal nutrition across mammalian species has led to more detailed studies on mechanisms, using the mouse Emb‐LPD model. These studies have shown that programming of the embryo to maternal Emb‐LPD has occurred by the blastocyst stage. This has been demonstrated by transferring blastocyst stage embryos to control‐diet foster mothers, which does not prevent the persistence of programming consequences (Watkins et al. 2008a). Analysis of maternal serum and uterine fluid composition has indicated that Emb‐LPD reduces insulin and amino acid levels during the period of preimplantation development which may be influential in the induction of embryo programming (Kwong et al. 2000; Eckert et al. 2012). Individual analysis of amino acids has shown specifically that the concentrations of branched‐chain amino acids (BCAAs), leucine, isoleucine and valine, are up to 30% reduced in Emb‐LPD uterine fluid at the time of blastocyst morphogenesis (Eckert et al. 2012). These metabolites are known mediators of cellular growth and protein translation through the mTORC pathway (Wang and Proud 2009). Indeed, blastocysts collected from Emb‐LPD mothers exhibit both significantly altered pools of free amino acids and reduced mTORC signalling compared with control blastocysts from mothers fed a normal diet, strongly implicating this nutrient pathway in embryo ‘sensing’ of maternal dietary status (Eckert et al. 2012). The role of insulin and BCAAs in the induction of abnormal fetal growth and onset of cardiovascular disease has been further demonstrated in an in vitro model, which mimics the Emb‐LPD in vivo environment (Velazquez 2014). Following induction of embryo programming mediated through maternal Emb‐LPD, the embryo activates a series of compensatory responses within the extra‐embryonic lineages, first evident within the expanding blastocyst, to promote nutrient delivery to the conceptus despite the deficit in maternal nutrient quality. These responses appear critical in promoting survival and competitive fitness of the offspring and affect both (i) trophectoderm – chorio‐allantoic placenta and (ii) primitive endoderm – visceral yolk sac cell lineages. Within the trophectoderm, formed on the outer surface of the blastocyst and facing the uterine lumen, there is an increase in the proliferation rate (Eckert et al. 2012) as well as an increase in fluid‐phase and receptor‐mediated endocytosis and cytoplasmic vesicular organization for enhancing degradation of externally‐derived nutrients (Sun et al. 2014). This endocytosis response to Emb‐LPD includes upregulation of expression of the megalin endocytic receptor, increased presence of endocytosed vesicles and lysosomes and is regulated by Rho‐A GTPase acting on the actin cytoskeleton via mTORC2 signalling (Figure 16.2). Interestingly, we find that the inductive mechanism of adverse developmental programming by Emb‐LPD maternal diet mediated through low BCAAs (see above) is responsible for endocytic activation since this phenotype can be generated in vitro
The Embryonic Environment and Developmental Origins of Health
Introduction
In Vivo Environmental Condition and Embryo Plasticity
Overnutrition Models
Maternal Undernutrition Models
Consequences
Causes
Stay updated, free articles. Join our Telegram channel
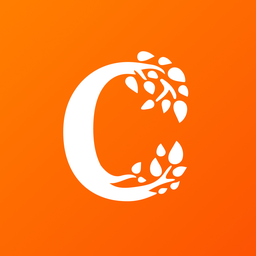
Full access? Get Clinical Tree
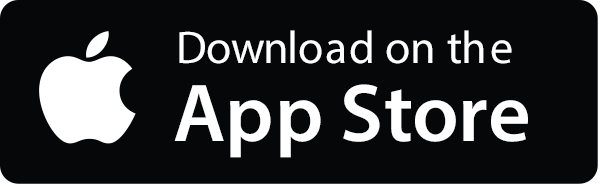
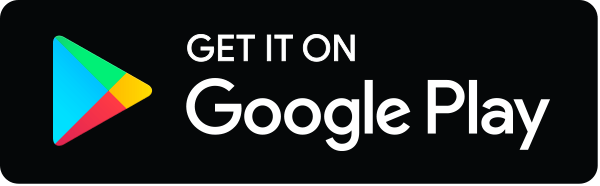