The ecology of Escherichia coli
David M. Gordon, The Australian National University, Canberra, ACT, Australia
The genus Escherichia
The genus Esherichia and the species E. coli have been recognized for over a century. In 1985, Farmer and colleagues described the first new species in the genus, E. fergusonii (Farmer et al., 1985). In 2003, Huys and colleagues described the second new species, E. albertii (Huys et al., 2003). Hyma et al. (2005) described the evolutionary relationship of E. albertii to E. coli, and its identity to the diarrheal pathogen, Shigella boydii serotypes 7 and 13. All of the other named species and serotypes of Shigella are actually members of E. coli (Sims and Kim, 2011). There are three other named species of Escherichia: E. blattae, E. vulneris, and E. hermannii, but strains of these species are only distantly related to other Escherichia and are not valid members of the genus (Walk et al., 2009).
The techniques of multi-locus sequence typing (MLST) and multi-locus sequence analysis (MLSA) have revolutionized our understanding of the evolution, ecology, epidemiology, and population genetics of bacteria (Maiden et al., 1998). There are three MLST schemes used for E. coli and MLST data have been collected for a great many isolates (http://mlst.ucc.ie/mlst/dbs/Ecoli, www.pasteur.fr/recherche/genopole/PF8/mlst/EColi.html, and http://www.shigatox.net/stec/cgi-bin/index). Coupled with the growth of the MLST databases, there are an increasing number of studies that isolate and characterize E. coli from non-clinical sources (Gordon, 1997; Souza et al., 1999; Pupo et al., 2000,b; Gordon and Cowling, 2003; Power et al., 2005; Wirth et al., 2006; Walk et al., 2007), and as a consequence our understanding of the diversity of bacteria has increased enormously. These studies have revealed substantially more genetic variation in the genus Escherichia, and five ‘cryptic clades’ of Escherichia were described (Walk et al., 2009). The term cryptic clades was used because, based on standard phenotypic methods, strains belonging to these novel Escherichia species are phenotypically indistinguishable from E. coli. Our current understanding of the relationships among the various Escherichia lineages is illustrated in Figure 1.1.
FIGURE 1.1 This phylogeny presented depicts our current understanding of the diversity to be found in the genus Escherichia and the phylo-group structure observed with the species E. coli. The phylogeny also demonstrates that both major types of E. coli pathogens, those causing diarrheal disease (EPEC, ETEC, STEC, EAEC, and Shigella; in red) and extraintestinal infection (ExPEC; in blue) are present in multiple phylogenic lineages. Strain names are presented as well as ST numbers (MLST hosted at http://mlst.ucc.ie/mlst/dbs/Ecoli) when available and the pathovar designation for the strain. Strains in black were isolated from the feces of asymptomatic hosts.
E. fergusonii has been implicated as an opportunistic extraintestinal pathogen of humans (Farmer et al., 1985; Funke et al., 1993; Savini et al., 2008), birds, and mammals (Herráez et al., 2005; Hariharan et al., 2007). It has been suggested that E. fergusonii is also capable of causing intestinal disease (Bain and Green, 1999; Chaudhury et al., 1999), but the evidence for this supposition is weak. Genes associated with extraintestinal disease (e.g. papG, sfa/focG, cnf1, ibeA, fyuA, iroN, and ompT) are lacking in E. fergusonii, as are intestinal disease factors such as astA, sat, pic, stx1, and eaeA. By comparison, E. albertii is a known diarrheal pathogen of humans (Huys et al., 2003; Oaks et al., 2010; Ooka et al., 2012), an avian pathogen responsible for epidemic mortality in finches in Alaska and Scotland (Foster et al., 1998; Oaks et al., 2010), and has been implicated in the death of other bird species (Oaks et al., 2010). All strains of E. albertii appear to possess the intimin locus and produce cyto-lethal distending toxin B (Oaks et al., 2010). Escherichia strains belonging to the cryptic lineages CI–CV encode a variety of extraintestinal and intestinal virulence factors, sometimes at high frequencies (Ingle et al., 2011). However, with the exception of clade I strains, there appears to be little evidence to suggest that they are potential pathogens. Enterotoxigenic E. coli (ETEC) appear to have evolved on multiple occasions and Steinsland et al. (2010) identified one clonal group of ETEC strains, CG12, that they estimated to be atleast ten times older than all other ETEC clonal groups. ETEC CG12 strains are members of cryptic clade I suggesting that at least some members of this clade are potential diarrheal pathogens.
Where does E. coli occur?
E. coli can be isolated from plants and a wide variety of animals. While E. coli can be recovered from ectothermic vertebrates it is more frequently encountered in homeotherms (Gordon and Cowling, 2003). However, even among birds and mammals, factors such as body size and gut morphology are important predictors of the likelihood of isolating E. coli from a particular host (Gordon and Cowling, 2003). In birds and carnivorous mammals the probability of detecting E. coli in a host increases with the body size of the host. This outcome is likely a consequence of the relationship that exists between body mass and gut transit times. For example, in the carnivorous marsupials (Dasyuridae) gut transit times vary from about 1 hour for the 18-g Sminthopsis crassicaudata to 13 hours for the 1000-g Dasyurus viverrinus. Gut morphology also appears to play a role. Insectivorous bats have relatively short undifferentiated tube-like intestines that lack a cecum, while a similar-sized rodent has a well-differentiated intestine and possesses a cecum. E. coli is much less frequently isolated from bats than from similar-sized rodents. Experiments with rats fed diets differing in the concentration of crude fiber resulted, as expected, in food transit times through the gut declining with increasing fiber concentration (O’Brien and Gordon, 2011). These experiments also demonstrated that E. coli cell densities declined with decreasing transit times. Extrapolating the observed linear relationship between cell density and gut transit time predicts that E. coli should not be found in animals such as bats (O’Brien and Gordon, 2011). A variety of other factors may also influence E. coli cell densities in a host. For example, pregnancy coupled with excessive weight gain (Santacruz et al., 2010) or starvation in children (Monira et al., 2011) may elevate E. coli cell densities. Many different antibiotics have also been shown to lead to significant increases in E. coli cell density (Looft and Allen, 2012). However, there are little specific data on the nature of the interactions occurring between the host, E. coli, and other members of the gut microbiota.
Host effects, gut morphology and dynamics, and the gut microbiota are not the only factors determining the likelihood of detecting E. coli in a host. Background levels of contamination are also important. E. coli is more likely to be recovered from frogs, reptiles, or birds living in association with humans than in those living in undisturbed habitats such as national parks (Gordon and Cowling, 2003). The climate where the host lives also appears to be a factor in determining whether E. coli can be detected in a mammalian host. In Australia, E. coli is unlikely to be detected in hosts living in the desert and less likely to be detected in hosts living in the tropics, compared to hosts living in temperate regions of the country (Gordon and Cowling, 2003).
Genetic structure of E. coli
It has long been recognized that there is considerable genetic substructure in E. coli (reviewed in Chauduri and Henderson, 2012). In addition to the well-known phylo-groups A, B1, B2, and D, there is phylo-group E of which O157:H7 is the best-known member. Other workers recognize the existence of phylo-groups known as C and F (Tenaillon et al., 2010). Phylo-group C strains are closely related to phylo-group B1 strains and phylo-group F strains are related to D and B2 strains. Strains of the cryptic clade I should also be considered a phylo-group of E. coli (Luo et al., 2011). The relationships among the different phylo-groups of E. coli are depicted in Figure 1.1. Although there is a well-established and reliable PCR-based method of determining the phylo-group membership of an E. coli isolate (Clermont et al., 2000; Gordon et al., 2008), MLST is the only method, at present, capable of identifying strains belonging to phylo-groups C, E, and F.
Numerous studies have demonstrated that the distribution of strains belonging to the phylo-groups A, B1, B2, and D is very non-random. For example, among Australian vertebrates, strains belonging to phylo-group B1 are most frequently isolated from frogs, reptiles, birds, and carnivorous mammals such as bats and quolls, whilst B2 strains are rare in such hosts (Figure 1.2). Among humans living in developed countries such as Australia, the United States, and Europe, B1 strains are less often encountered than strains belonging to the other phylo-groups (Figure 1.3). By contrast, phylo-group A and B1 strains appear to be predominant in humans living in developing countries (Figure 1.3).
FIGURE 1.2 Relative abundance of the main E. coli phylo-groups (A, B1, B2, D) with respect to the source of isolation. Unpublished data and data taken from Gordon and Cowling (2003), and Power et al. (2005).
FIGURE 1.3 Relative abundance of the main E. coli phylo-groups (A, B1, B2, D) with respect to the source of isolation. Data taken from Unno et al. (2009), Tenaillon et al. (2010), and Li et al. (2010).
Consequently, the morphology and dynamics of the gastrointestinal tract as well as host diet appear to influence the phylo-group membership of strains present in a host. There is some experimental evidence to support these conclusions. B2 strains occurred at lower cell densities in rodents fed diets high in crude fiber as compared to the cell densities they achieved when the crude fiber concentration of the diet was low (O’Brien and Gordon, 2011). Diet effects may explain the very different relative abundances of the phylo-groups recovered from humans living in different parts of the world.
Therefore, strains belonging to the different phylo-groups appear to have different ecological niches and life-history characteristics. Phylo-group A and B1 strains appear to be generalists able to occupy a broad range of vertebrate hosts. By contrast, B2 and D strains are more commonly isolated from birds and mammals than ectotherms. It has been argued that phylo-group B2 strains are the most host adapted as, at least in humans, there are data to suggest they persist longer in a host than strains of the other phylo-groups (Nowrouzian et al., 2005, 2006). They are also competitively dominant, as hosts harboring a B2 strain have, on average, fewer detectable E. coli genotypes than hosts harboring strains belonging to other phylo-groups (Moreno et al., 2009). There is also a growing body of evidence to indicate that the transmission dynamics of strains of the different phylo-groups also differs. For example, phylo-group A and B1 strains are over-represented in freshwater samples, whilst B2 and D strains are rare in such samples (Power et al., 2005; Walk et al., 2007; Ratajczak et al., 2010). The persistence of E. coli strains in soil also varies with their phylo-group membership (Bergholz et al., 2010). A phenotype commonly linked to a strain’s ability to survive stressful conditions is the red dry and rough (rdar) phenotype, where cells produce an extracellular matrix composed of curli fimbriae and a variety of polysaccharides, is more frequent in B1 strains than in strains belonging to the other phylogroups (White et al., 2011).
While it is clear that strains belonging to the different phylo-groups of E. coli differ in their phenotypic, ecological and life-history characteristics, there is little understanding of the underlying mechanisms leading to these differences. To a very great extent this difficulty has arisen because of the enormous genetic diversity to be found in E. coli (Touchon et al., 2009). A typical E. coli genome consists of about 4700 genes, however, only about 2000 of these genes are common to all E. coli strains. The balance of the genes in the genome of a strain is drawn from a gene pool that is in excess of 10 000 unique genes, after eliminating all transposable elements and prophages. To date, there has been no systematic attempt to determine whether or not any genes are over-represented in a particular phylo-group.
Strains of the various phylo-groups do vary in their phenotypic properties, such as carbon source utilization patterns (Gordon, 2004) and their ability to cause disease in a mouse model of extraintestinal infection (Johnson et al., 2006). Strains of the various phylo-groups also differ in their gene content, with phylo-group B2 strains in particular harboring a variety of traits, often at high frequency, thought to enhance the ability of a strain to cause extraintestinal disease. These same traits have been shown to be important determinants of a strain’s ability to colonize the intestine (Diard et al., 2010).
The hierarchical structure of E. coli extends beyond the species or phylo-group level to clonal complexes and clonal lineages (MLST sequence type (ST) or clonal complex (CC)) (Figure 1.1). Researchers studying E. coli responsible for intestinal infections have long recognized the significance of particular clonal groups, for example, the infamous O157:H7 clonal group (ST 11). Particular clonal lineages are also often responsible for extraintestinal infections such as the phylo-group D clonal group A strains (ST 69) (Johnson et al., 2011); or the phylo-group B2 strains O1:K1:H7/NM (ST 95) (Mora et al., 2009). Other lineages are less often responsible for extraintestinal infection, but very commonly isolated from the feces of humans and other animals. The group of strains related to E. coli K12, belonging to ST 10, is the best known of these as it represents a very large fraction of the sequence types in the E. coli MLST database hosted by University College Cork.
Strains responsible for extraintestinal infection may originate from any of the E. coli phylo-groups, although most isolates are members of phylo-groups B2 and to a lesser extent, D. All of the available evidence indicates that the main pathotypes responsible for intestinal disease have arisen from each of the phylo-groups, often on multiple occasions (Figure 1.1). Although for the intestinal pathogens, phylo-group A, B1, D, and E strains were more likely to have provided the ancestral lineages than phylo-group B2 strains (Escobar-Páramo et al., 2004; Yang et al., 2007). It is often also the case that a particular pathogenic lineage has as its closest relative a non-pathogenic variant (Figure 1.1).
Within and among host E. coli diversity
Studies based on non-selective plating (e.g. MacConkey) of fecal E. coli isolates have revealed that typically one or two genotypes are recovered per host, although as many as six or more may be detected (Caugant et al., 1983; Alm et al., 2011). However, such studies seldom use sample sizes that permit the detection of genotypes occurring at a frequency of less than 5% of the total E. coli population. Selective plating, usually for antibiotic-resistant variants, almost always reveals the presence of additional genotypes that represent less than 1% of the total E. coli population (Gordon et al., 2002). E. coli have been isolated from different regions of the small and large intestines of domestic pigs and wild boars (Dixit et al., 2004; Schierack et al., 2009). These studies have revealed that the focus on fecal isolates underestimates the diversity of isolates present in the gut. A similar outcome has been observed for O157:H7 in cattle, where rectal swabs can result in significantly higher frequencies of positive animals than do fecal isolations (Naylor et al., 2003). Significantly, the pig studies have shown that there are isolates detected in the small intestine that are not detected in the colon or feces. The reasons for this outcome are unknown but probably reflect the fact that cell densities achieved in the small intestine are from 100 to 1000 times lower than those achieved in the colon. Further, there is experimental evidence to suggest that non-adherent E. coli cells do not divide (Poulsen et al., 1995). Hence non-adhering cells originating in the small intestine would be significantly outnumbered in the feces. Although there are very limited data, strains from different gut regions appear to have different characteristics. For example, bacteriocin production was less frequent among isolates from the duodenum as compared to isolates from downstream regions and the kinds of bacteriocins produced differed between strains isolated from the ileum and those taken from feces (Abraham et al., 2012). Food transit times are more rapid in the upper small intestine and nutrient concentrations high compared to the lower intestinal tract (Timm et al., 2011). Theoretical studies indicate that bacteriocin producers should be disadvantaged under these conditions compared to non-bacteriocin producers (Frank, 1994; Barnes et al., 2007).
As a first approximation it may be stated that every human harbors a different numerically dominant strain of E. coli. In a study of fecal isolates recovered from 228 people, it was found that only 27% of the observed clones were recovered from more than one individual (Johnson et al., 2008). However, the sharing that did occur was highly structured. Strain sharing was observed in 313 of the total potential sharing pairs, however 27% of within-household pairs shared a clone, while only 0.8% of across-household pairs did.
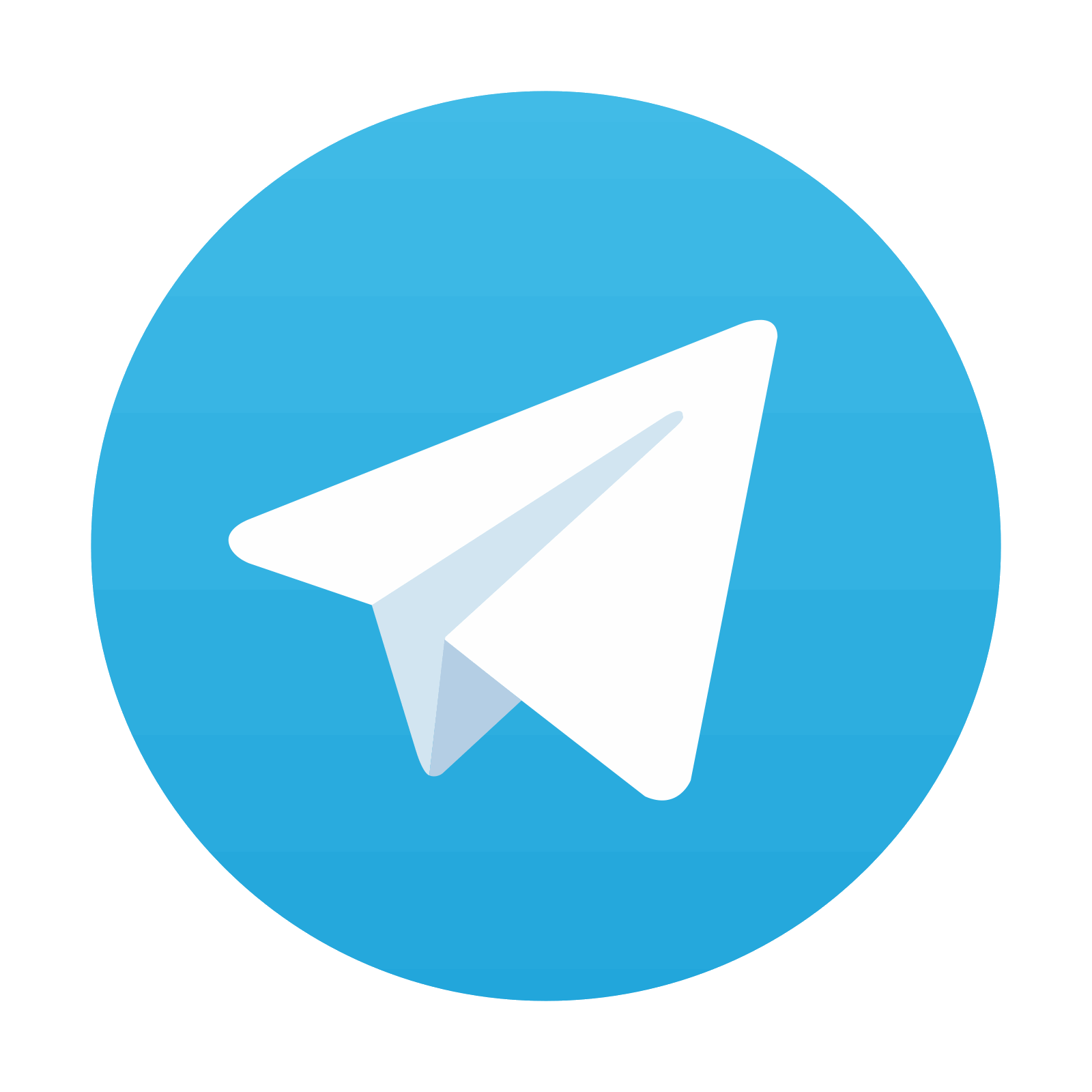
Stay updated, free articles. Join our Telegram channel
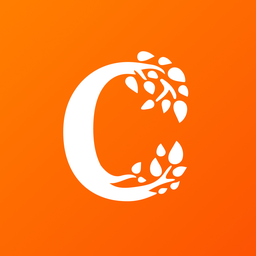
Full access? Get Clinical Tree
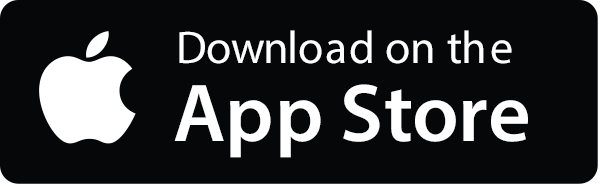
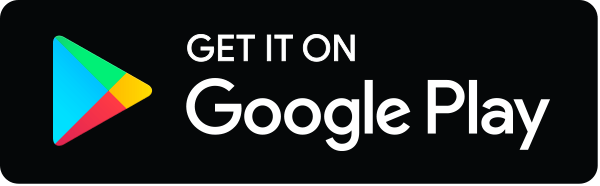