and Miguel A. R. B. Castanho2
(1)
Instituto de Bioquímica Médica Leopoldo de Meis, Federal University of Rio de Janeiro, Rio de Janeiro, Rio de Janeiro, Brazil
(2)
Institute of Biochemistry and Institute of Molecular Medicine, School of Medicine, University of Lisbon, Lisbon, Portugal
Our idea of the interior of a cell at the molecular scale is often rather naïf. If one could see the interior of a cell with molecular resolution, one would not see an aqueous solution of molecules with the cellular organelles suspended. The molecular crowding, in particular the macromolecular crowding, inside a cell is such that the interior of a cell is more like a gel than a solution. Molecular packing is so dense that it is hard for macromolecules to diffuse freely. The ubiquitous presence of the cytoskeleton and macromolecular assemblies in a space that is highly restricted due to cellular organelles makes the interior of cells tightly packed (Fig. 2.1). Nevertheless, it is a highly hydrated environment, where solvation is made by water molecules (Fig. 2.1) and voids are filled by water that solubilizes ions and small molecules. Thus, virtually all exposed molecules in a cell are under the chemical and physical influence of water. The interior of a cell is not an aqueous solution, but the chemical reactions of the living cells are typical chemical reactions of aqueous solutions.
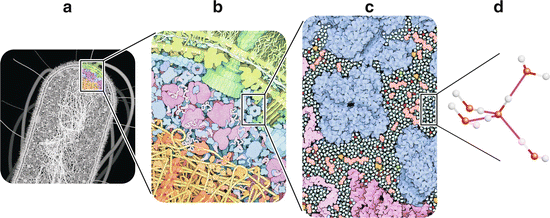
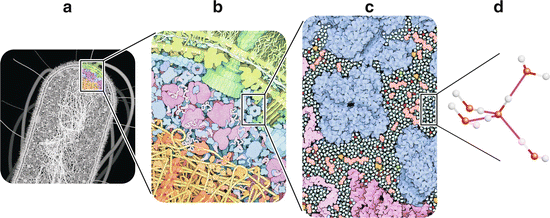
Fig. 2.1
Crowding in the molecular organization of cells. Even in simple cells such as bacteria (e.g., E. coli , a), cytoplasm is a dense tight packing of macromolecules (e.g., proteins and nucleic acids) and smaller molecules such as water, nucleotides , and amino acid s (b). This situation is frequently referred to as “molecular crowding.” The cell interior is thus more similar to a gel than to a solution. Nevertheless, virtually all the external surface of macromolecules, small molecules, and ions are in direct contact with water (c). Amino acids, saccharides , ATP , and many other small organic molecules are shown in pink. Metal ions are represented in red, phosphate ions are yellow and orange, and chloride ions are in green. Water molecules are colored turquoise. Although in many cases water molecules may be confined to the solvation shells (immediate layers of water surrounding other molecules), they impose many chemical and physical constraints to molecular organization and reactivity in cells. Water molecules are polar and prone to establish hydrogen bonds (d). Although the cell interior is not a solution, its chemistry and physics is dominated by water. Panels (a–c) are reproduced with permission from Goodsell, The Machinery of Life, 2009
Life started in water and, chemically speaking, it is still dominated by water. Even the elemental composition of the molecules in a cell was determined by water. With few exceptions, the abundance of elements in a cell reflects the abundance of the same elements in the oceans (Fig. 2.2). Iron, phosphorus, or nitrogen is among the exceptions: they are more abundant in cells on average than in sea water due to their so-called chemical utility. The electronic structure of nitrogen (Fig. 2.2) makes it appropriate as an electron donor to establish dative covalent bonds, also known as dipolar or coordinate bonds. Phosphorus is an amazing element for its capabilities in coordination chemistry (Fig. 2.2). The chemistry of phosphate, PO4 3−, is so useful to cells that phosphorylation /dephosphorylation is an ubiquitous mechanism to activate or inhibit enzymes or determine the reaction a molecule will undergo in a metabolic sequence. Iron may have once been abundant as soluble Fe2+ that was afterward oxidized to Fe3+ upon the appearance of molecular oxygen , O2, in the Earth’s atmosphere. Fe3+ formed oxides and hydroxides that precipitated, making iron less abundant in seawater. Nonetheless, iron was already being used by cells, and its “chemical utility” determined that cells kept this element at higher levels in their composition. Iron is able to participate in coordination chemistry organometallic complexes, hemoglobin being an example.
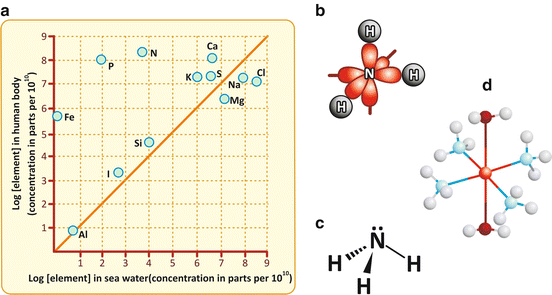
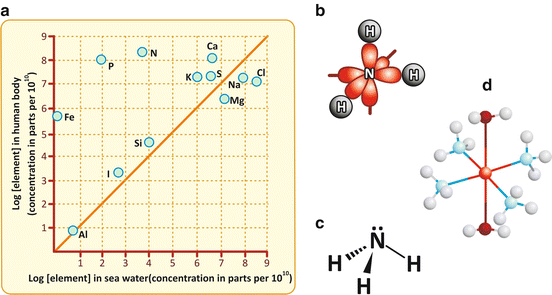
Fig. 2.2
Panel (a) shows that the abundance of the chemical elements in the human body matches the abundance of such elements in seawater, with few exceptions (reproduced with permission from Dobson et al., Foundations of Chemical Biology, 2001). Iron, phosphorus, and nitrogen are among such exceptions because they are very abundant in the human body. Elements such as carbon, hydrogen, and oxygen are not represented in the graph because they are extremely abundant. Nitrogen binds three hydrogens in ammonia (NH3). The covalent bonds are formed with the s orbitals of H (gray) and a mix of the s (not shown) and the three p orbitals of N (red) (b). A fourth mixed sp orbital has electrons that are available to participate in dative (coordination) bonds. A simplified representation of the molecule represents these electrons as •• over N (c). Oxygen in water or dioxygen (O2) molecules also have electrons available to participate in dative bonds, for instance, with metal ions. Panel (d) shows the tetraamminediaquacopper(II) cation, [Cu(NH3)4(H2O)2]2+, in which a central Cu2+ ion accepts electrons from two water and four ammonia molecules. Binding of dioxygen to iron in hemoglobin follows the same principle
Elements that are very abundant on Earth’s crust but not in the oceans such as aluminum (8.2 % of the atoms) or silicium (28 % of the atoms!) are also not abundant in cells because they are mostly part of insoluble oxides. Only 1 % of the total atoms in cells are not hydrogen (62.8 %), oxygen (25.4 %), carbon (9.4 %), or nitrogen (1.4 %). Yet, many elements that are only present in trace amounts may be part of molecules or processes essential to life, such as boron (B), cobalt (Co), cupper (Cu), manganese (Mn), or molybdenum (Mo).
So, water imposed severe constraints on the molecular evolution of cells. And still does! Water is a small but amazing molecule. In spite of its simplicity and abundance, water still fascinates chemists. In particular, its polarity and ability to establish hydrogen bonds are determinant to influence chemical reactions in regions where water serves as a major solvent, which means almost the whole cell (lipid membranes are the main exception). If it weren’t for hydrogen bonding, for instance, water would not be a liquid at temperature and pressure ranges humans and most living beings are adapted to. Methane, with a molecular structure that can be compared to water, is not as nearly important as water in the history of life: methane boiling point is −162 °C (Fig. 2.3).
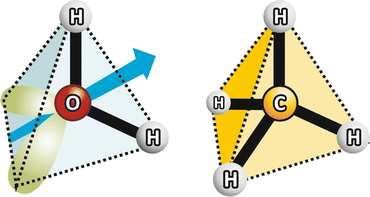
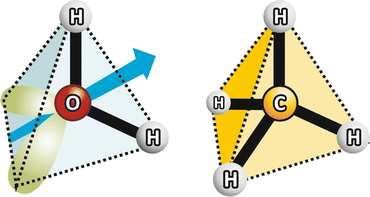
Fig. 2.3
Water vs. methane: similar molecular geometry but different polarity (charge asymmetry axis represented in blue from the slightly higher electronic density in the nonbonding O orbitals in green to lower electronic density in H) and different hydrogen bonding capabilities, which determines very different ebullition points: 100 °C to water, −162 °C to methane
Molecules that are polar, mainly those that can establish hydrogen bonding with water, can be distributed in the human body by simple diffusion, such as in blood and/or cerebrospinal fluids, while nonpolar molecules have low solubility and can only be distributed by special carrier systems, such as lipoproteins (see Sect. 3.1.2). Glucose (Fig. 2.4), for instance, distributes in the human body without a carrier, as its concentration in blood can reach very high values with no solubility problems. Cholesterol is the opposite as its solubility in aqueous environment is very low, so it would form crystals and precipitate if it was not kept in nonpolar environments such as the hydrocarbon core of lipid membranes or the interior of lipoproteins. Some pathological situations such as gallstones relate to the low solubility of cholesterol -related molecules, which leads to formation of aggregates (due to the so-called entropic effect; see Sect. 3.1) that in turn nucleate small crystalline structures that grow into “stones” that can measure up to a few centimeters.
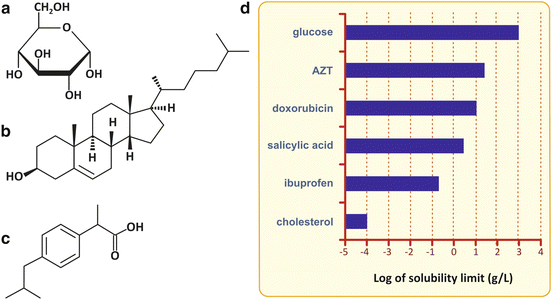
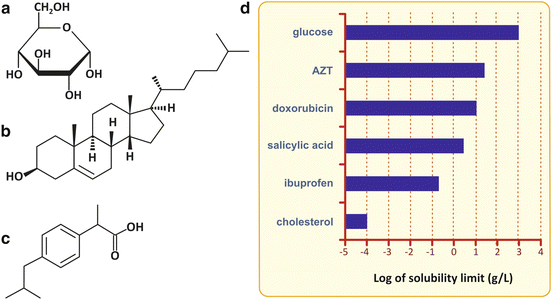
Fig. 2.4
Very hydrophilic molecules are polar, such as glucose (a). Like water, glucose can establish several hydrogen bonds per molecule. Its solubility in water is therefore very high, and glucose can be distributed freely in the human body when solubilized in the blood plasma. In contrast, cholesterol (b), having only a single polar group, the alcohol (–OH), is very hydrophobic (nonpolar) and is not soluble in aqueous media, such as blood plasma. Distribution of cholesterol and other nonpolar molecules in the human body requires special structures that emulsify these hydrophobic molecules in the blood, the lipoproteins. Some biological molecules and drugs, such as ibuprofen (c), for instance, may be intermediate cases and have limited solubility due to the simultaneous presence of hydrophobic and hydrophilic groups in the same molecule (ibuprofen has a phenyl ring and a carboxylic group), which is very important for their ADME : absorption, distribution, metabolization, and excretion by the human body. Solubilities of selected biological molecules and drugs are presented in (d)
Solubility is as important for pharmacology as it is for physiology. A drug that precipitates and crystallizes in the blood, or even in the stomach after being swollen, is hardly effective as it cannot be absorbed and/or distributed in the body. Nonpolar drugs are usually mixed with other molecules in formulations that prevent drug aggregation in aqueous environment. Solubility is one of the key parameters considered to devise drug development strategies.
The tendency that drugs have to locate in aqueous or hydrophobic regions of tissues, such as lipoproteins, lipid bilayers, or adipose depots, is studied in pharmacology by measuring the partition of the drug between octanol and water, two immiscible solvents. Octanol is an organic solvent, largely apolar. In spite of the popularity of this method, octanol in still hardly similar to lipidic environments and more modern alternatives exist, like working with aqueous suspensions of lipid vesicles (Fig. 2.5).
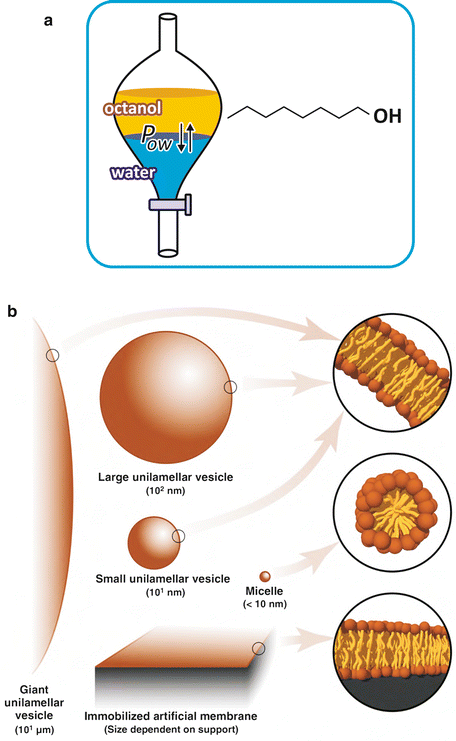
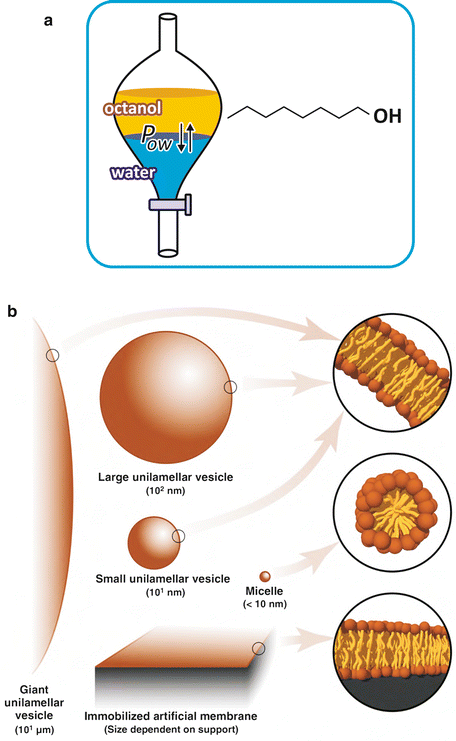
Fig. 2.5
(a) Octanol is an amphiphilic molecule with an acyl chain of eight carbons and a polar alcohol group (–OH). Its hydrophobicity prevents its miscibility with water. Depending on their own polarity, solutes will distribute more extensively to octanol or water. The ratio of the equilibrium concentrations of the solute in both phases is constant, regardless of the total amount of solute or the volume of each phase, which is the reason why this ratio is referred to as partition constant, P ow. This parameter is used to estimate the tendency molecules under study (e.g., a drug candidate for a future medicine) have to interact with membranes and other lipid ic structures, such as lipoproteins or lipid droplets. (b) More recent techniques use different approaches (artificial lipid bilayers in suspension). Despite the ubiquity and simplicity of the octanol/water approach, octanol is a poor replica of lipids . Lipid vesicles in suspension are lipid bilayers of very well-defined composition. Although they lack many characteristics of biological membranes (receptors, transporters, cytoskeleton anchorage, clustering of specific lipids, etc.), they are much more realistic as biological membrane mimetics than octanol. Figure b reproduced from Ribeiro et al., Trends Pharmacol Sci 31:449–454, 2010, with permission of Elsevier
The polarity of a given molecule is also determinant for its excretion. Polar molecules are easier to excrete for their solubility in blood and urine. The main strategy of the human body to eliminate xenobiotics (molecules that are not natural constituents of human tissues) consists in grafting hydroxyl (OH) groups, so that they become more polar and, therefore, more soluble in aqueous fluids. This is an efficient method that can be applied to a wide diversity of molecules, serving the purpose of low specificity for a broad protection of the human body against multiple toxic molecules. The molecular complex responsible for polyhydroxylation of different compounds is cytochrome P450 , a big proteic complex that contains iron (Fig. 2.6).
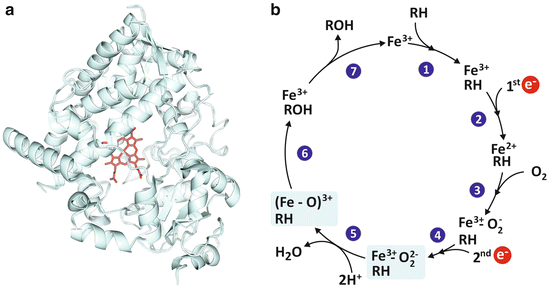
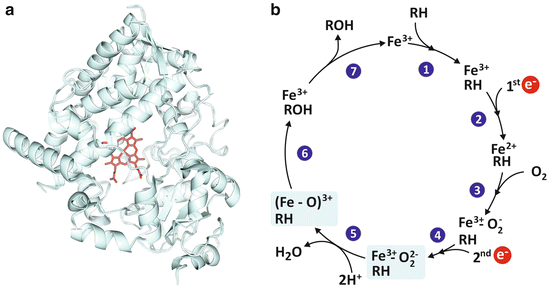
Fig. 2.6
The P450 cytochrome complex (a; PDB 1W0E) uses iron and oxygen to add an hydroxyl (OH) group to organic molecules (generically represented by RH in panel b) rendering it more water soluble, suitable to be excreted through urine. This is an important detoxifying mechanism in the human body
Hydrophobicity (more accurately one would say the “entropic effect”) is important not only for the absorption, distribution, and excretion of molecules, as discussed in this section, but also for holding together the most ubiquitous of all supramolecular non-covalent structures of cells: the lipid bilayer membranes . The importance of this issue is such that it will be kept for detailed explanation in a later section (Sect. 3.1)
2.1 The Basics of Chemistry in Cells and Tissues
The boundaries between chemistry and physics at the molecular scale are hard to establish. The interface between both is a rich scientific field referred to as physical chemistry or chemical physics. These disciplines deal typically with molecular structure and the way reactivity is affected by it. A functional distinction that is very practical for those working with molecules is to consider chemical reactions of all transformations of matter that involve formation or breakdown (or both) of covalent bonds. Transformations that do not involve alteration of covalent bonds are considered physical processes. Thus, light being absorbed by the molecules on the skin surface by a protective “sunscreen” cream constitutes a physical process, while UV radiation reaching the skin cells and damaging DNA due to covalent bond cleavage is chemistry. On a macroscopic scale, this functional frontier between chemistry and physics may lose intuitive sense: a plumber cutting a metallic or PVC tube would then be doing chemistry as he is actually destroying chemical bonds in doing so.
In practice, a clear definition of what is chemistry by opposition to what is physics is not needed or useful. Many professionals use both and do not really mind or think about naming what they are doing in terms of chemistry vs. physics classification.
The “chemical life” of cells is very rich and diverse. Many different kinds of reactions may occur. Probably all kinds of reactions occurring in aqueous medium that are described in the most complete organic chemistry textbooks can be found in cells. In this chapter, we will focus only on those most important to understand human metabolic regulation, the current core of human integrative biochemistry (i.e., biochemistry in relation to other disciplines such as histology, physiology, pharmacology, and even anatomy, so that a global perception of human body homeostasis is achieved). It should be stressed that such reactions are favored by the presence of water as solvent. As interaction with solvent molecules affects the electronic distribution of molecules, their reactivity is affected by the solvent. If natural evolution at molecular level was based on a different solvent (e.g., octanol), the “portfolio” of the chemical reactions of life would be different.
Oxidation–reduction reactions are among the most important reactions of the living world. As the name implies, oxidation–reduction reactions are those in which electrons are donated (oxidation) or received (reduction). Because electrons in cells do not remain isolated, individually, as they would in vacuum in outer space, they are transferred between molecules or ions and therefore oxidation and reduction coexist. They are thus referred to as oxidation–reduction reactions. Many different molecules may be oxidized or reduced. Some are particularly well adapted as reducing agents, such as NADH (Fig. 2.7); others are particularly well adapted to be part of a chain of successive electron transfers, such as some metalloproteins. Metalloproteins have metallic elements in their composition that facilitate reception and donation of electrons. The electron transport system, for instance, has several of these proteins (see Sect. 6.2.3).
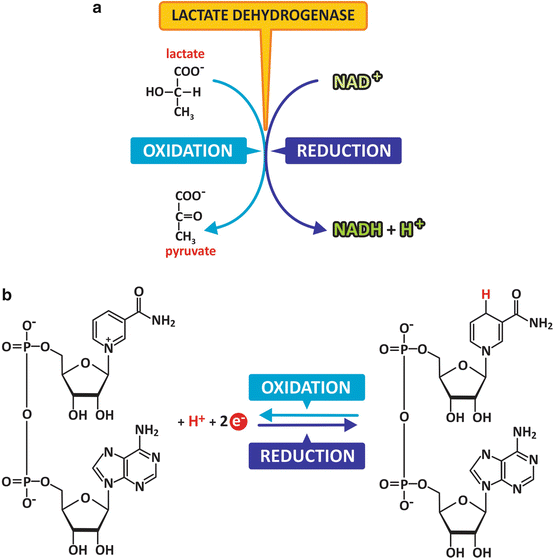
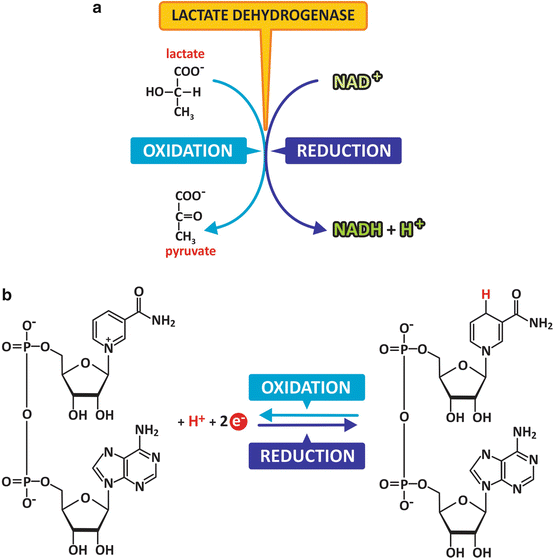
Fig. 2.7
Example of an oxidation–reduction reaction. Lactate is oxidized to form pyruvate (a). Its –OH group is transformed in a C=O (carbonyl) group, and electrons were transferred to NAD+ in the process, which was transformed in NADH (b)
Acid–base reactions constitute another class of extremely important and ubiquitous reactions in the “living world.” These are reactions in which a proton (H+) is either donated (by an acid) or received (by a base). In aqueous environment, such as in the almost totality of the cell, H+ does not exist as such because water molecules capture the proton forming H3O+ or donate a proton, forming OH−, the hydroxide anion. These chemical species (OH−, H3O+, and H2O) are all related and equilibrium among them may be reached:
The equilibrium constant for this reaction is:
Because [H2O] (molar concentration of water) is constant at any given temperature and pressure, the so-called ionic product of water, K w, is used instead for its simplicity:
Nearly at 25 °C, K w = 1 × 10−14 mol2 dm−6 (i.e., 1 × 10−14 M2). This may seem rather pointless at a first glance, but it is from here that one can conclude that in pure water, pH value is 7. In pure water one ion of H3O+ is formed for each OH−, therefore:
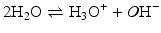
![$$ {K}_{\mathrm{eq}}=\frac{\left[{\mathrm{H}}_3{\mathrm{O}}^{+}\right]\left[{\mathrm{O}\mathrm{H}}^{-}\right]}{{\left[{\mathrm{H}}_2\mathrm{O}\right]}^2} $$](/wp-content/uploads/2016/11/A215639_1_En_2_Chapter_Equb.gif)
![$$ {K}_{\mathrm{w}}=\left[{\mathrm{H}}_3{\mathrm{O}}^{+}\right]\left[{\mathrm{O}\mathrm{H}}^{-}\right] $$](/wp-content/uploads/2016/11/A215639_1_En_2_Chapter_Equc.gif)
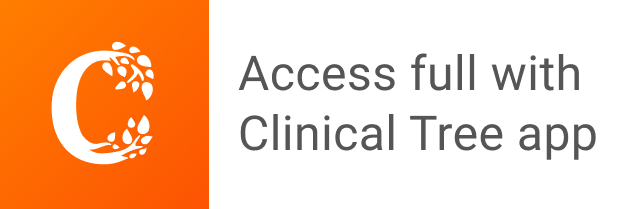