The Changing Face of Innovation in Drug Discovery
Bristol-Myers Squibb Company, Princeton, NJ, USA
Introduction
Over the past 30 years, pharmaceutical companies have contributed many innovative medicines for the treatment of human diseases. Scientific and technological advances have enabled the discovery of important drugs in critical areas such as infectious diseases, cancer, cardiovascular disease, and metabolic disorders such as diabetes. Since 1982, the U.S. Food and Drug Administration (FDA) has approved about 500 new chemical entities (NCEs) [1]. Among these, more than 100 drugs [2–5] work through new mechanisms of action (Table 4.1). Our knowledge, facilitated in large part by advances in genomics and a plethora of new approaches aimed at uncovering the essential facts of cellular and physiological pathways, may finally be delivering on its promise, as evidenced by the significant increase in first-in-class drugs between 2003 and 2012 (Table 4.1). Cancer drug discovery, for example, has evolved rapidly, thereby shifting the focus toward targeted medicines with improved efficacy and safety. The field of immuno-oncology [6, 7], which targets the immune system in certain types of cancer, holds great promise, as illustrated by the approval in 2012 of the first true immune-oncologic, Yervoy [8], which has been bringing great hope to many melanoma patients. Biopharmaceutical research has also witnessed increased efforts across the industry in the discovery of drugs intended for small populations of patients with rare (or “orphan”) diseases. These efforts were highlighted in 2011 [3], with 11 of the 30 new molecular entities (NMEs) approved by the FDA going to first-in-class drugs having a rare disease as the primary indication. Clearly, pharmaceutical companies are leading the way in the discovery of novel medicines, with the United States accounting for 50% of first-in-class drugs discovered between 2004 and 2012 [1]. In 2012 alone, the FDA approved a total of 37 NMEs, an output unseen since the late 1990s. The most exciting result for the pharmaceutical industry is that about 28 first-in-class NMEs were approved by the FDA in 2012. These data clearly show that innovation is resurging across the industry.
TABLE 4.1. New Chemical Entity (NCE) Approvals from 1982 to 2012

The number of NME approvals is one measure of success for pharmaceutical companies. Yet a commercial reality that constantly challenges continued investment in drug research is that after thorough clinical testing and the meeting of the appropriate regulatory requirements for approval, only about 8–12 years of patent exclusivity typically remain following the launch of a new drug. Patent expiration in most cases leads to a rapid decline in profit, as generic companies essentially commoditize the innovations of pharma companies. Moreover, modern drug discovery operates in a relatively new reality: the essential requirement that new drugs need to be differentiated from available therapies if they are to have any promise of delivering a return on investment. Because differentiation can only be demonstrated relatively late in the drug discovery process via carefully controlled human clinical trials, an even higher bar is now set for discovery organizations to provide a data package that supports investment.
Modern drug discovery is proceeding in an environment that has been radically altered to accommodate the explosion of new biological information. Engineering advances are steadily opening up exciting new possibilities for innovations, which in turn are leading to new and more efficient ways to identify and qualify new drug candidates. Technological advances have become a great facilitator for testing new ideas and thus now play an essential role in driving drug innovation. New technologies have led to new challenges and to questions like this one: How do we test, assimilate, and apply new technologies in a manner that efficiently enables future drug discovery? The industry, in its rapid evolution, has encountered several pitfalls related to overpromising results from technology. A hard lesson learned is that no single approach, such as high-throughput screening (HTS) or combinatorial chemistry, will deliver simply on its own. On the contrary, an integrated approach to leveraging technology in a cross-functional fashion has proven to be one important way of generating data that, used appropriately, can positively impact both the capacity and the cycle time needed for discovering new drug candidates.
The drug discovery process entails a broad range of disciplines—from genomics and target discovery to the identification and optimization of lead compounds for preclinical testing. Innovative technologies play an important role in enabling the integration of those disciplines. Over the past two decades, most companies have invested a great deal of human as well as economic capital to deploy advanced technology platforms, and with varying degrees of success. For example, technologies related to automation, informatics, and high-throughput assays have all played important roles in creating better data faster—data that guide the iterative nature of drug discovery.
In this chapter, we will focus on three themes:
- highlights of the key technological innovations that have revolutionized the drug discovery process in recent years
- important lessons learned from the process of creating and applying new technologies, and
- new trends that will drive future innovations in drug discovery research.
Core Objectives of the Drug Discovery Process
The pharmaceutical industry started on its long evolutionary journey in the nineteenth century with essentially “accidental” discoveries of chemicals derived from tars or natural derivatives, chemicals that could be used to treat human diseases [9]. Drug discovery as a formal process did not become integral to the pharmaceutical industry until the early years of the twentieth century. In the ensuing years—especially in the past half-century—drug discovery has joined clinical development, manufacturing, and regulatory sciences to become one of the most fundamental and critical business units in the industry. This has happened as the principles of chemistry have been increasingly applied to problems beyond chemistry itself, as pharmacology—the medical and biological study of drug activity—has become a well-defined scientific discipline in its own right, and as clinical research has yielded in-depth knowledge of diseases and their treatment.
Today, although some aspects of drug discovery vary from one company to another, the basic processes are the same everywhere. These processes can be divided into four interconnected processes:
- Target identification and target validation focus on discovering and confirming molecular targets that play a fundamental role in both normal and disease states.
- Hit identification entails using the information derived from target identification and validation to discover multiple molecules active against potential therapeutic targets.
- Lead optimization is a process designed to help optimize potency (a function of how tightly the molecules interact with the target), improve selectivity (the degree to which a dose of a drug produces the desired effect against a specific target), and evaluate the safety of leading molecules by applying iterative cycles of “medicinal chemistry” efforts.
- Preclinical assessment is performed to select the best candidate molecules for clinical development based on diverse in vitro and in vivo data packages.
For several decades, as pharmaceutical companies have used target identification and target validation, hit identification, lead optimization, and preclinical assessment, they have continuously monitored and evaluated these processes to improve them and thereby close any gaps in drug discovery. Improvement has come in the form of innovative solutions. Figure 4.1 highlights some key technological breakthroughs that have significantly improved the quality and capacity of these core drug discovery processes. These innovative solutions have indeed improved the quality and capacity of the work performed by drug discovery organizations across the industry.

One of the key lessons we have learned is that science must set the direction for technological innovations in discovery. Thus, the next phases of innovation need to be based on the challenges we are facing today. This is because the drug discovery process is an extremely challenging endeavor due to the fact that numerous hurdles beyond activity and selectivity have to be overcome. Recent studies [10, 11] indicate that failure appears to be occurring most often in Phase 2/3 and primarily for reasons of toxicity and insufficient efficacy. During preclinical assessment, therefore, thoughtful integration of toxicology work plans is critical to understand drug development risks, develop appropriate levels of testing schemes, and identify high-quality drug candidates.
Effective solutions to improve drug discovery success rates depend on many factors. First and foremost, effective tools for predicting efficacy and toxicity are urgently needed. Indeed, the judicious selection of therapeutic modalities with high success probabilities has become the most critical task for the discovery organization. In summary, technological innovations for drug discovery processes must transition from simply targeting capacity gaps to searching for solutions that can produce high-quality data, provide enriched information, and establish better connectivity between in vitro and in vivo models.
Major Innovations in Technology Have Advanced Drug Discovery
Innovations in technological advancement for discovery have been driven by the need to close substantial gaps in discovery processes. This section shows how innovations targeted at these gaps have produced the breakthroughs that greatly enhance drug discovery processes.
Target Identification and Target Validation
The sequencing of the human genome represents the largest undertaking in the history of biological science, and the completion of the Human Genome Project (HGP) created a new landmark in the life sciences [12]. While the predicted strong impact from HGP may not yet be felt in daily clinical practice, that day is definitely accelerating toward us. For example, researchers have already identified single genes associated with a number of diseases, such as cystic fibrosis, Duchenne muscular dystrophy, neurofibromatosis, and retinoblastoma [13–16]. As research progresses, investigators will decode the disease-associated mechanisms that are caused by a single gene or multiple genes interacting with environmental factors.
More important and relevant, the HGP has transformed the way a great deal of research is done in the pharmaceutical industry. Understanding unknown protein function in the human genome is continuously enriching the druggable target landscape [17–20]. The best-known technological advancements, such as genomics [21, 22] and proteomics [23], have significantly enhanced the capability for target identification and validation in the industry. Moreover, the measurement of the differential expression of messenger RNAs and proteins in both diseased and normal tissues has become an important technique widely used in the drug-target phases of discovery research.
Among the breakthrough technology platforms used in genomics-based research are gene microarray chips [24] containing a collection of oligonucleotides. Today, Affymetrix chips [25] can be used to measure the expression of more than 10,000 human genes in a single experiment. Furthermore, microarray chips for the determination of gene expression in other species, such as rats, mice, Escherichia coli, Drosophila melanogaster, Caenorhabditis elegans, and Saccharomyces cerevisiae, are also commercially available. These tools significantly accelerate the identification of novel therapeutic target genes that are expressed differently in disease tissues and models. Yet the major challenge associated with this approach is that the number of “hits” is dependent on the magnitude of significant biological manipulations. As a result, the “hits” are typically limited to hundreds of genes. Furthermore, those changes at the mRNA level cannot always be automatically translated into corresponding alterations in protein expression activity in the disease states. Nonetheless, the next gene sequence technologies [26, 27] have enabled us to identify mutations in the abnormal genes in disease states accurately and rapidly. The applications of next-generation sequencing (NGS) technologies and gene microarray chips have opened a possible channel for personalized medicine by determining whole-genome and gene function information just-in-time.
To facilitate target identification and target validation, discovery research has been increasingly turning in the past 5 years or so to the task of converting DNA sequence information into disease-associated protein functions. Analysis of a proteome—the entire set of proteins expressed in an organism, cell, or organelle—is very challenging because protein functions are in constant flux. Proteomics has to deal with the separation of highly complex protein mixtures. In addition, the difference in concentration between low-abundance and high-abundance proteins can exceed more than five orders of magnitude; hence, proteins in high abundance can mask those in low abundance. This situation leads to big challenges in protein detection and protein separation. To help meet this challenge, innovative advances in liquid chromatography–mass spectrometry (LC-MS) technology [28, 29] have transitioned proteomics from identifying functional proteins to illuminating quantitative changes in key protein components within cellular systems. Quantitative analysis of global protein levels, termed “quantitative proteomics,” [23] is important to the system-based understanding of both the molecular functions and molecular mechanisms of each protein component in the various biological processes and systems.
Although genomic and proteomic approaches have provided more target options, so far the pharmaceutical industry has only investigated a subset of human genome encoding ∼800 proteins [30]. It is estimated that at least 3000 druggable targets are in the human genome [31, 32]. The overlap space between the disease-relevant human genome pool and drug-like molecules [17–20] is referred to as druggable space [19] (Figure 4.2). Remarkably, medicinal chemists working over the decades and across industry have made millions of diverse chemical structures. However, only about 1000 of these molecules have been approved as drugs. Currently, the great challenge related to druggable space is that the “low-hanging fruit” among targets has largely been picked, and the druggable space has begun to shrink. Thus, to expand the druggable genome and accelerate drug-target discovery processes, new and innovative target discovery approaches have to be explored and applied.

In recent years, chemogenomics [33–36] has become an increasingly important approach to target identification. With this approach, large collections of compounds are screened for parallel identification of biological targets and biologically active compounds. The success of the chemogenomics approach depends on three critical components: (1) a compound library, (2) a representative biological system (a target library, a single cell, and a whole organism), and (3) a reliable readout (e.g., gene/protein expression, high-throughput binding, or a functional assay).
An example of the application of chemogenomics is to identify novel and disease-associated G-protein-coupled receptors (GPCRs) [33, 37], which constitute a large and diverse family of proteins whose primary function is to transduce extracellular stimuli into intracellular signals. GPCRs represent one of the most important drug-target families because more than 50% of the current therapeutic agents on the market target these receptors [38, 39]. In the human genome, over 800 GPCRs [40] have been identified. Yet more than half of these have sensory functions and are generally not relevant for drug discovery. Moreover, only 300 GPCRs are considered druggable [19, 40]. Approved drugs and ongoing clinical trials account for about 100 out of 300 GPCRs. Today, there are still over 100 nonsensory orphan druggable GPCRs [41, 42]. So it is not surprising that across the drug discovery industry, there is a great deal of interest in exploring novel GPCRs.
Chemogenomic approaches can be used for novel GPCR target discovery for three main reasons. First, due to the important role that GPCRs play in drug discovery, diverse GPCR compound libraries have been designed and generated at most of the leading pharmaceutical companies [43]. The major sources of GPCR compound collections are based on (1) the homology models derived from the rhodopsin crystal structure [44], (2) the use of site-directed mutagenesis in relation to ligand structure–activity relationships (SARs), and (3) the accumulated knowledge from past and ongoing clinical compounds. The GPCR compound collections at pharmaceutical companies have become one of the critical drivers in the contemporary chemogenomic environment.
Second, our knowledge of GPCR functions in biological systems is no longer limited to the isolated receptor level. In fact, the dynamic functions of GPCRs in a cellular context have been extensively investigated. It is well known that more than half of all drugs target GPCRs by either activating or inhibiting GPCR activity. The binding of specific ligands—such as hormones, neurotransmitters, chemokines, lipids, and glycoproteins—activates GPCRs by inducing or stabilizing a new conformation in the receptor [45–47] (Figure 4.2a). Activated receptors (R*) can then activate heterotrimeric G proteins (composed of α.GDP, β, and γ subunits) on the inner surface of the cell membrane. The GPCR signaling process is subsequently triggered by a specific ligand β that binds and activates the receptor, thereby inducing a conformational change in the receptor. The receptor, thus activated, stimulates a specific G protein by catalyzing the exchange of guanine nucleotides in the α-subunit. The GTP-bound α-subunit and the βα subunits disassociate from the receptor and activate their respective downstream GPCR effector proteins. Unfolding GPCR signaling pathways provide researchers with molecular insights into the key intermediates lying between ligand-mediated cell surface receptors and biological responses (Figure 4.2b). As a result, diverse biological systems can be used today to identify novel GPCR targets. Physiologically relevant systems, such as primary cells and tissues, have become the leading option for identifying novel GPCRs and building connectivity between targets and disease states via chemogenomic approaches.
Finally, high-throughput technology platforms [48–51] have laid a solid foundation for measuring GPCR compound activities in the high-throughput mode at the receptor level, G-protein level, and G-protein-dependent second messenger level (Figure 4.2b). Phenotypic technology platforms, such as high-content image systems and label-free platforms, have provided powerful detection systems for chemogenomic approaches to support GPCR target research [52–54].
Phenotypic screens play a critical role in target identification and validation. The concept of a phenotypic screen is not new; in fact, phenotypic screening approaches have been used in the drug discovery business for decades. A recent review paper [4] provides an in-depth analysis of NMEs approved between 1999 and 2008. The data demonstrate that during this period, 45 approved drugs were first-in-class, and out of those drugs, 27 (60%) were discovered using phenotypic assays, and 18 (40%) were discovered using target-based approaches. These data clearly illustrate the important role of phenotypic screening.
Phenotypic approaches do not require prior understanding of the molecular mechanism of action, but they can reflect compound activity in physiologically relevant systems. Therefore, they can be translated into a given disease state more effectively than the nonphenotypic approaches, target-based assays, which are often more artificial. The main disadvantage of phenotypic screening approaches lies in the challenge of optimizing the molecular properties of candidate drugs in the absence of target-mechanism knowledge. The sensitivity and reproducibility of phenotypic screens can also form a bottleneck in the process of assessing target–activity relationships (TARs) precisely. In any case, the integration of phenotypic screening approaches and target-based assay technologies will bring about a future paradigm shift in target identification and target validation [55–57].
Hit Identification: The First Stage in Drug Hunting
For every discovery program, the entry point is the identification of specifically active molecules—“hits”—that have adequate activity in a suitable target assay. Such initial hits can be generated in a number of ways. One of the common approaches is HTS [58–61]. Today, HTS is defined as the process of testing batches of compounds for binding activity against relevant disease targets. Since the 1980s, innovations and enhancements in screening technologies have yielded remarkable results. About 30 years ago, the annual number of throughputs was a about 10,000 compounds. Today, the daily number using ultrahigh-throughput screening approaches is more than 100,000 compounds—a staggering 1000-fold increase. The increase can be ascribed to a number of important technological innovations.
At present, HTS assays are typically performed on “automation-friendly” microtiter plates with a 96-, 384-, or 1536-well format [58]. The remarkable transition from a Petri dish to a 1536-microtiter plate reflects over a century of evolution in drug discovery screening approaches. Figure 4.3 shows the path that innovations in basic lab equipment have taken over in the past 100 years. Such innovative solutions have made today’s low-cost and rapid HTS assays possible.

The rapid expansion and maturation of HTS in the relatively recent past is a good example of how scientific needs drive technology innovations in the pharmaceutical world. As suggested earlier, there was, in the early 1990s, a rapidly growing need to perform many biochemical or cellular assays on many compounds in an efficient and quantitatively rigorous manner. At that time, however, screening thousands of compounds in a single day presented an impossible task. Researchers at pharmaceutical companies soon realized that the gaps in drug screening required that the industry work closely with equipment and software vendors to develop a wide range of enhanced technologies. The industry’s willingness to champion cutting-edge technology platforms gave vendors the incentive to take risks in researching, developing, and licensing powerful new products. The exciting cooperation between pharmaceutical companies and vendors resulted in a wide spectrum of hardware and software innovations that allowed the lofty HTS goals to be met and, in time, surpassed.
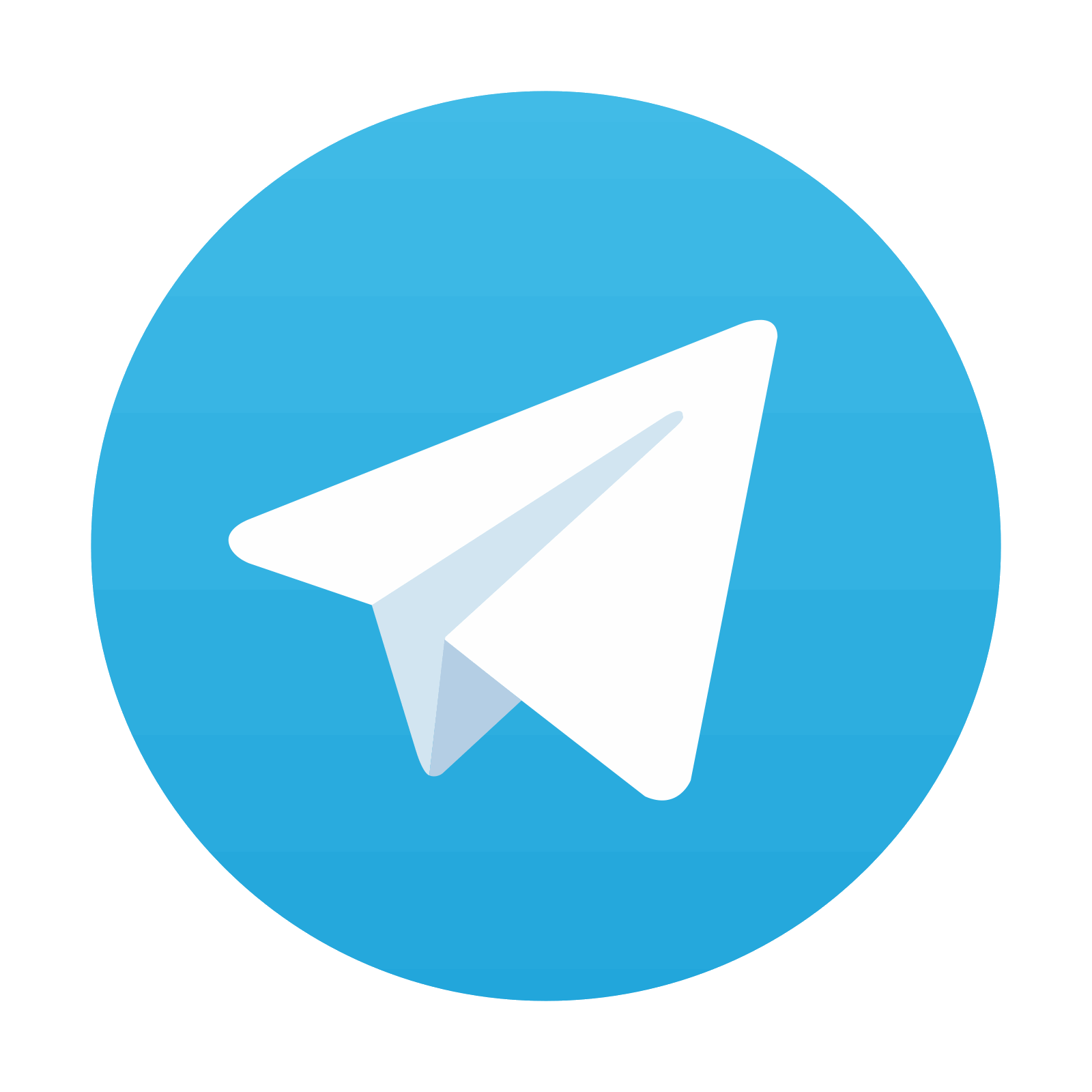
Stay updated, free articles. Join our Telegram channel
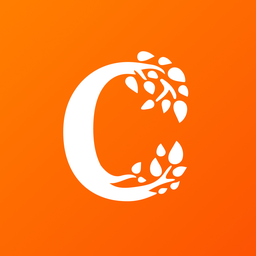
Full access? Get Clinical Tree
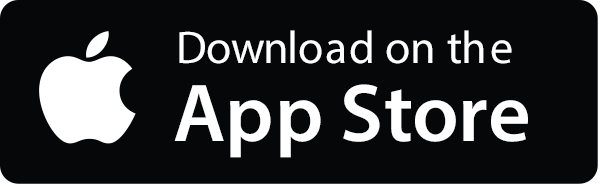
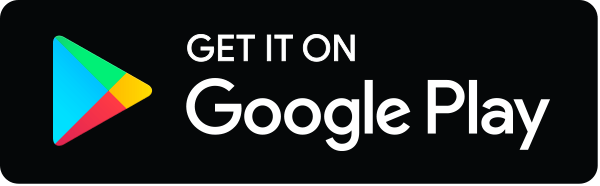