Finding the Middle Ground: Drug Discovery Technology in the Era of Academic Screening Centers
BioTechniques, New York, NY, USA
Since the early 1990s, using high-throughput (HT) chemical screens for the purpose of lead compound discovery has been the realm of the pharmaceutical industry. As small molecule libraries expanded in size and end point assays increased in complexity, HT lead discovery screens required the use of an increasingly sophisticated array of large-scale robotics systems, small well volume plates (either 384- or 1536-wells), rapid liquid transfer and plate washing devices, and high-content imaging instrumentation capable of reading cellular phenotypes. However, the overall results of this brute force, HT approach are questionable. The number of lead compounds in late-stage clinical trials has not significantly risen in the past ten years, leading to smaller pharmaceutical drug pipelines even at a time when older drugs are coming to the end of their patent protection life [1]. However, a paradigm shift in the manner by which chemical screens are performed and by whom they are initiated has occurred in recent years [2]. This shift is due in large measure to the emergence and continuing expansion of academic chemical screening facilities that possess expertise and technological sophistication on par with major pharmaceutical centers. The number of academic screening centers around the world has continued to rise for the past five years, and the trend does not appear to be temporary. In 2009, the Society for Biomolecular Sciences Reference Library listed 64 screening centers in their Academic Screening Facility Directory. However, by late July 2011 that number had risen to 84 facilities according to the Society for Laboratory Automation and Screening directory.* Such a steady growth rate over a short period of time is most certainly due in part to the increasing availability of advanced screening technologies, instrumentation, and chemical libraries that can be applied at varying levels of throughput depending on the specific needs of an investigator or center—key elements for academic screening facilities. In this chapter the recent evolution of academic screening centers will be examined, with a specific focus on the effects technology and methods developments are playing in shifting the priorities of researchers when it comes to their chemical screening efforts. In addition, the possible roles that academic screening facilities might play in the development of drugs in the coming decades will also be explored.
Large-Scale Screening Centers and Lead Compound Discovery
Increasing availability, not to mention flexibility, of HT robotic systems and screening technologies has enabled several academic centers to implement chemical screening platforms with throughput and capacity on par with their pharmaceutical counterparts [2]. However, there are differences: due to the needs of academic screening centers, the ability to perform a wide range of screening applications (both in terms of compound library size and assay design) on a particular platform is critical as different investigators using the facility will have differing needs (size of screen, end point assay, etc.) when performing their particular HT screen.
Since the implementation of their chemical biology platform, the Broad Institute located in Cambridge, Massachusetts has been involved in a wide array of chemical screening projects. For example, in 2009 Gupta et al. explored the possibility of identifying inhibitors of epithelial cancer stem cells using a HT screening approach [3]. Following the development of an assay demonstrating that breast cancer cell populations induced into epithelial–mesenchymal transition were indistinguishable from cancer stem cell populations, the authors screened a small molecule library of 16,000 compounds containing both commercially available small molecules and natural extracts; they identified 32 compounds that exhibited toxicity toward the induced breast cancer cells [3]. Although only a subset of the Broad Institute’s complete chemical collection of nearly 500,000 compounds [4], studies such as this provide an example of how proper assay design with a smaller collection of molecules can provide new biological insights and possible lead compounds for clinical testing—another key factor for academic centers, where it is crucial to keep both screening time and costs as low as possible.
The varied nature of screens performed at academic centers can be seen in a somewhat larger screen that was also conducted at the Broad Institute chemical biology platform searching for novel antimicrobial agents. Here Moy et al. [5] screened the nematode Caenorhabditis elegans for survival when infected with the human pathogen Enterococcus faecalis. The authors designed an assay wherein infected worms were placed into individual wells of 384-well plates using a large particle sorter. Live animals were screened for increased survival with 37,200 small molecules and natural product extracts using the dye SYTOX Orange, which only enters cells with damaged membranes [6]. The screen resulted in the identification of 28 compounds not previously reported to have antimicrobial effects, and gave further insight into the cellular mechanisms of action of these antimicrobial agents.
As more academic facilities enter into the world of chemical screening, the need to understand and minimize the sources of error from HT screening (i.e., false-negative and false-positive results [7]) has never been greater. This concern has led some researchers to develop HT screening applications wherein the numbers of false positives or false negatives could potentially be decreased significantly. In 2006, Inglese et al. reported a quantitative HT screening approach where greater than 60,000 compounds were titrated in series in 1536-well microplates [8]. Using low-volume dispensing, high-sensitivity detectors, and robotic plate handling, the authors were able to generate concentration-response curves to determine structure-function relationships in a screen of pyruvate kinase modulators. The ability to generate such dose-response curves for such a large number of samples and small molecules, thereby minimizing false negatives within an HT screen, is a direct result of the development of automation technologies that enable the more widespread use of 384- or 1536-well plates.
HT screens undertaken now at centers such as the Broad Institute are being accomplished using technologies that, although similar in design, differ from what has been previously applied in pharmaceutical settings. Several academic screening facilities have invested in and utilize “modular designs” for their screening instrumentation to enable a diverse array of screening assays and applications to be performed. For example, instrument development companies such as HighRes BioSolutions have constructed screening platforms where key instrumentation is placed on carts that can be moved or connected (“docked”) for specific assays. Such approaches increase the potential configurations of the screening platform (although some elements in these configurations, including the larger robotic arms, are fixed), therefore enabling a greater number of possible screening scenarios. Larger instrumentation, including imaging systems such as the FLIPR Tetra from Molecular Devices, can also be added when called for in a specific assay.
While pharmaceutical companies and larger academic screening centers might perform 60 or more HT screening campaigns in a given year [2], these are not the only players now when it comes to chemical screening; smaller centers are also on the rise around the globe. For example, with a compound library collection of 55,000 small molecules and natural products, the University of California at Santa Cruz chemical screening center is capable of screening up to 30,000 compounds per day, relying on technologies including PerkinElmer Janus systems for liquid handling, a BioTek plate washer, a Matrix Wellmate stacker, and various imaging systems, including Molecular Devices ImageExpress for automated image analysis to accomplish their goals.*
Chemical Genomics
The availability of commercial systems to perform HT screens, as well as interaction among scientist in different disciplines, is fueling the continued expansion of another form of chemical screening, the chemical genomic, or chemogenomic, screen. Chemogenomic screens seek to determine a drug’s effect by exploring the treatment of that drug on a set of different strains or isolates [9].
Here yeast has proven a fertile ground in which to apply chemogenomic screens owing to the generation and availability of large yeast deletion collections [10]. Such collections can be used to screen compounds and generate fitness profiles that can then be compared and used as a starting point to identify gene relationships [11]. In their review, Hoon et al. describe the various approaches, including forward screens such as haploinsufficiency profiling, homozygous profiling, and multi-copy and overexpression-suppression profiling, as well as reverse approaches such as toxicity-suppression profiling, that have been developed to either define the mechanism of action of a drug on a yeast biological process or identify a particular drug target. As noted in the previous section, cost is always an issue and chemical genomics screens using large numbers of strains and/or small molecules can be cost prohibitive and labor intensive, not to mention that in many cases there is only a limited amount of compound available to test. To overcome these issues, Pierce et al. developed a chemogenomic approach for yeast using the so-called TAG4 barcoding array that resolves fitness profiles for individual yeast strains that have been pooled by extracting changes in the abundance of the individual strains measured using the barcodes. Thus, the approach reduces the physical screening numbers as well as the volume of compound used in each screening assay [12].
Chemogenomic screens do have the ability to reveal novel insights into the mechanism of action of a particular compound. However, the ability to derive meaningful insights from such a chemical genomics screen is critically dependent upon developing the proper assay. A 2010 study by Kapitzky et al. demonstrates that crossing species boundaries when performing fitness profiling can provide such novel insights [9]. Here the authors created a screening platform using libraries of Saccharomyces cerevisiae and Schizosaccharomyces pombe haploid deletion mutants. By screening 727 S. cerevisiae and 438 S. pombe strains against 21 compounds, a DNA damaging agent that had a conserved mode of action with human cells was identified.
Chemogenomic screens are not exclusively used with organisms (such as yeast) where large collections of deletions mutations have been generated. A more recent example of the value that can be derived from performing chemical genomic profiling in other organisms can be see in the work of Yuan et al. and their profiling of antimalarial therapies and molecular targets [13]. Here, Yuan and colleagues from the National Institutes of Health Chemical Genomics Center and Columbia University attempted to both (1) identify new drugs with activity against Plasmodium falciparum, the malaria parasite, and (2) understand the mechanism of drug activity and resistance at the molecular level. The rationale for performing such a chemical genomics screen in this case is that resistance to antimalarial drugs has been demonstrated to occur (i.e., chloroquine resistance) and a more complete understanding of the underlying molecular mechanisms of drug activity could be beneficial in predicting or even eliminating such resistance in the future.
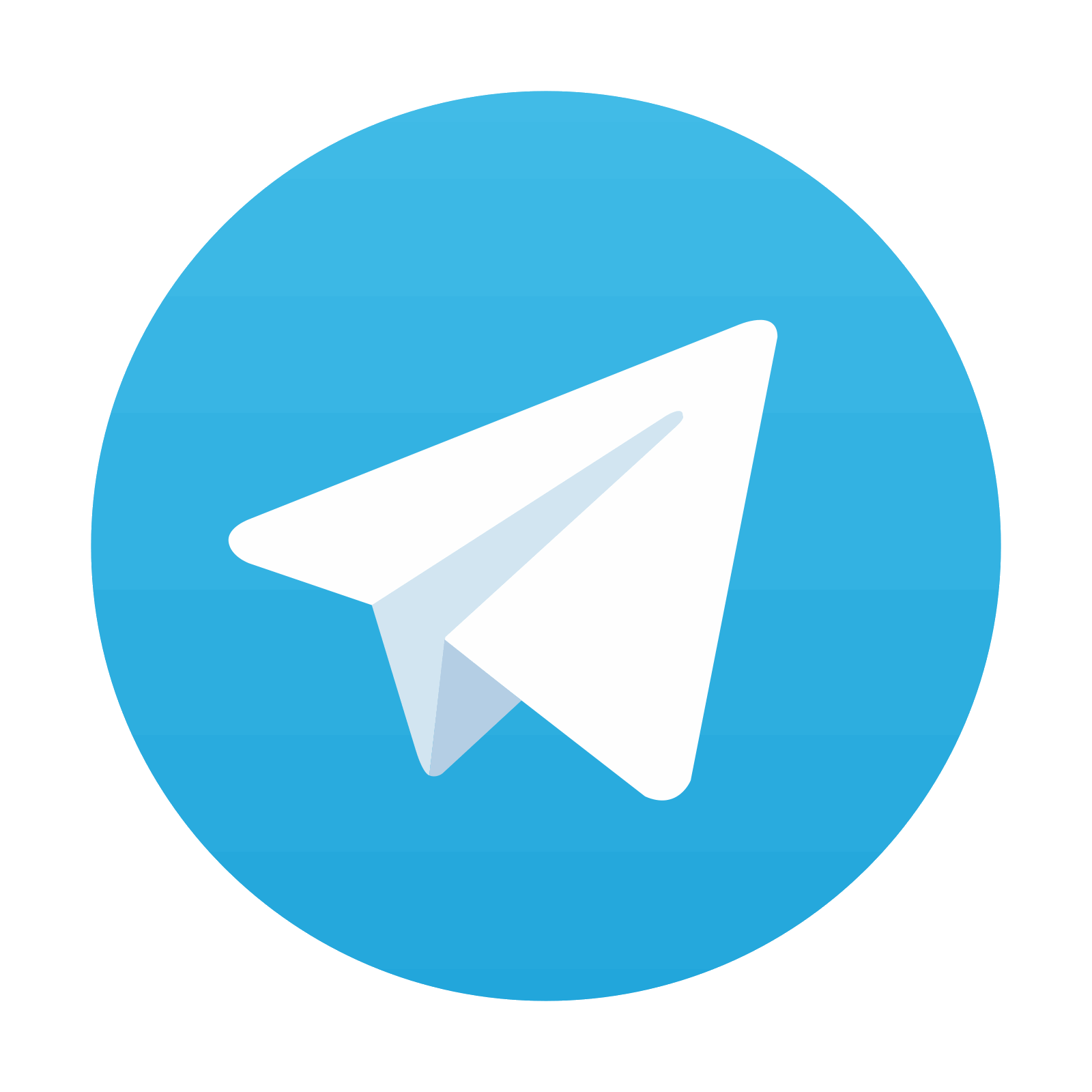
Stay updated, free articles. Join our Telegram channel
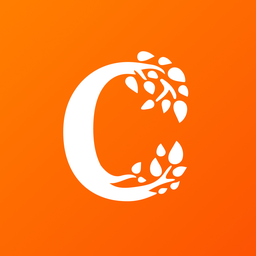
Full access? Get Clinical Tree
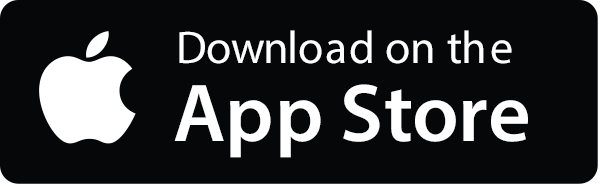
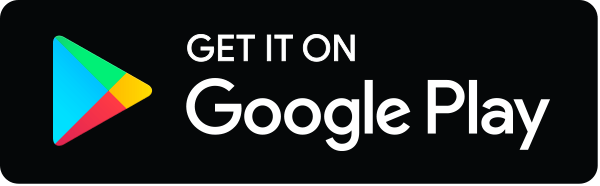