Fig. 5.1
Collagen Biosynthesis: It involves synthesis of pro-α and β chains, self-assembly of pro-collagens, secretion, cleavage of pro-peptides and self-assembly into fibril
Decorin (Li et al. 1992), lumican (Blochberger et al. 1992; Kao et al. 2006), keratocan (Chakravarti 2006; Corpuz et al. 1996), and mimecan (Funderburgh et al. 1997) are the four major proteoglycans that regulate the stromal collagen fibril formation and its stabilization. These corneal proteoglycans belong to the small leucine-rich type proteoglycan gene family. The polypeptide chain of the core protein is coiled into a tight spiral with a leucine-rich region located at every 360°, thereby aligning all the leucine-rich regions on one surface of the coil. These aligned leucine-rich regions interact with the collagen molecules to regulate fibril formation (Hassell and Birk 2010).
The core protein of the corneal stromal proteoglycans, namely, decorin, has a single 55–60 kDa chondroitin/dermatan sulfate chain, while lumican, keratocan, and osteoglycin/mimecan have 2–3 keratan sulfate chains of 10–15 kDa each, that regulate the spacing between the fibrils. Keratan sulfate (KS) is an important constituent of several collagen-rich tissues and is the major glycosaminoglycan (GAG) in the cornea, where it is N-linked to asparagine residues on the core protein by the corneal Glc-NAc 6-O-sulfotransferase enzyme (Akama et al. 2001). The sulfate groups of the keratan sulfate are essential to maintain the hydration level in cornea and for the solubility of the proteoglycan. Mutations resulting in defective proteoglycans lead to corneal dystrophies (Yasutaka Hayashida, PNAS 2006).
5.1.3 Endothelium: The Pump and the Barrier Function
The inner most layer of the cornea is the endothelium that forms a uniform monolayer of hexagonal cells (5 μ thick) with a cell density of around 2500 cells/mm2. The endothelium functions as both a barrier to fluid movement into the cornea and an active pump to move the fluid out of the cornea, ensuring corneal transparency. The barrier function was first demonstrated in the nineteenth century by Leber (Stocker and Reichle 1974). The function, as metabolic pump, discovered almost a century later in 1972, when Maurice DM (Freeman 1972) demonstrated that the endothelium possessed active, energy-dependent fluid pump that moved water from the corneal stroma into the anterior chamber. This ensures corneal transparency. The number of endothelial cells is therefore critical (at least around 400–500/mm2) for the optimal pump activity. Steady-state hydration occurs when the endothelial pump rate equals the GAG-driven leak (Maurice et al. 1981). A “pump-leak” mechanism where the active transport properties of the endothelium represent the “pump” and the stromal swelling pressure represents the “leak” was established (Riley 1969a, b). For the pump to function, Na+/K+-ATPase activity and the presence of HCO3 −, Cl−, and carbonic anhydrase activities are required. The pump requires Na+/K+-ATPase activity that actively transports Na+ from the stroma to the anterior chamber and forms a diffusing gradient for water, thereby maintaining a constant stromal hydration. Several basolateral (stromal side) anion transporters, apical (facing the aqueous humor) ion channels, and water channels have been identified. Numerous anion transport mechanisms have been identified in the endothelium, namely, basolateral Na(+)/2HCO(3)(−) cotransport, Na(+)/K(+)/2Cl(−) cotransport, Cl(−)/HCO(3)(−) exchange, and apical anion channels permeable to both Cl(−) and HCO(3)(−), apart from the carbonic anhydrase-mediated CO2-diffusive mode of apical HCO3 − flux and apical Ca2+-sensitive chloride channel (Bonanno 2003, 2012). The barrier function of the endothelium is in a dynamic regulation where the tight junctions and the adherent junctions play an integral role in addition to the Pumps and the channels (Srinivas, 2010).
Cellular glucose uptake is promoted by the glucose transporters present on both the apical and basolateral endothelial cell membranes (Kumagai et al. 1994). Eighty-five percent of glucose consumed in the cornea is converted to lactic acid (Riley 1969a). Epithelium is impermeable to lactate, and therefore in the cornea, it is about twice the concentration (~13 mM) to that in the aqueous humor (~7 mM), resulting in a large gradient for lactate flux from cornea to anterior chamber across the endothelium. Lactate: H(+) cotransport was demonstrated in the endothelium (Giasson and Bonanno 1994) (Fig. 5.2).
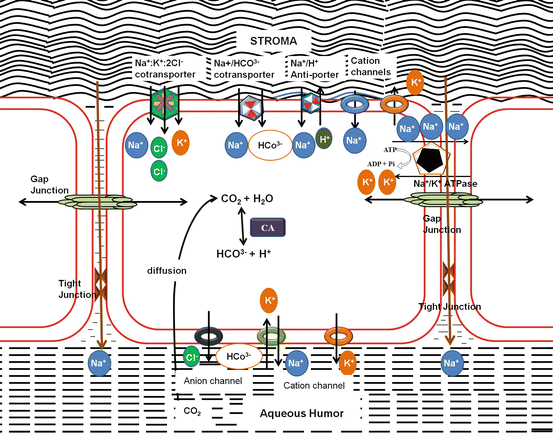
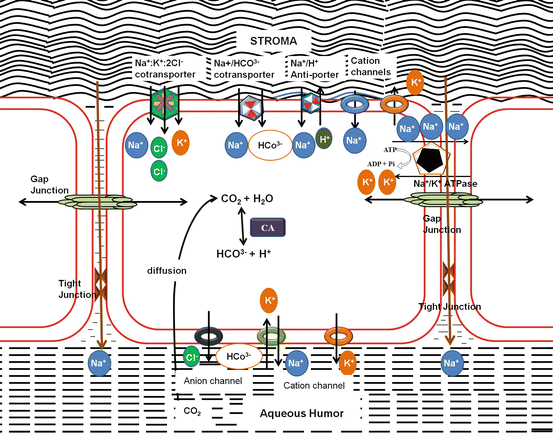
Fig. 5.2
Pump Leak Mechanism of stromal hydration: Stromal hydration is maintained by the pumps in the endothelium, ATP-driven Na+/K+ ATPase; Na+:K+:2Cl− co-transporter; Na+/HCO3− co-transporter; Na+/H+ anti-porter; selective and nonselective cationic and anionic channels
Corneal endothelium has a high density of aquaporin (AQP1) located on both apical and basolateral membranes (Kuang et al. 2004). AQP1 is present for the passive movement of the water for faster stromal hydration as in physiological situations such as eye closure (which swells the cornea by ~4 %) or exposure to hypo-osmotic conditions as in swimming (Bonanno 2012).
There is no mitotic activity of the corneal endothelial cells as these are inhibited in the G1 phase. There is 0.3–0.6 % loss of endothelial cells per year which is compensated by the migration of the neighboring cells or spreading to a pleomorphic shape. Cell loss is critical for the pump activity and can result in corneal edema, bullous keratopathy, and the loss of visual acuity. Cell loss occurs in trauma, refractive surgeries, penetrating keratoplasty, corneal dystrophies, diabetes, and glaucoma. Corneal endothelial dystrophy is a chronic progressive endogenous degeneration of the corneal endothelium, partly due to genetic predisposition (Joyce 2012). Therefore, current researches explore the proliferative capacity of these corneal endothelial cells.
The corneal endothelial dystrophies are congenital hereditary endothelial dystrophy 1 (CHED1), congenital hereditary endothelial dystrophy 2 (CHED2), posterior polymorphous corneal dystrophy (PPCD), and Fuchs endothelial corneal dystrophy (FECD) (Weiss et al. 2008). However, the molecular pathologies remain largely unknown. Genetic factors, chronic oxidative stress, mitochondrial damage, extracellular matix changes, endoplasmic reticulum (ER) stress changes, and apoptosis contribute to the pathology of FECD. Early- and late-onset FECD are distinct corneal conditions with the similar clinical and phenotypic characteristics but different genetic risk factors (Schmedt et al. 2012). Two types of congenital hereditary endothelial dystrophy (CHED) have been described: CHED1, which is autosomal dominant, and CHED2, which is autosomal recessive. The major differences between CHED1 and CHED2 lie on the onset, mode of inheritance, genetic mutations, and associated conditions. Mutations of 25 types in different locations of the SLC4A11 gene that codes for BTR1 (bicarbonate transport-related protein) or NaBC1 are known (Schmedt et al. 2012). Genetic defects in SLC4A11 disrupt the fluid flux across corneal endothelium necessary for maintenance of healthy corneal hydration. However, the mechanism by which the mutation leads specifically to atrophy of corneal endothelium is still not clear (Hemadevi et al. 2008; Vithana et al. 2006). Posterior polymorphous corneal dystrophy (PPCD) is a rare nonprogressive disorder that affects corneal endothelium and the DM (Henriquez et al. 1984). Mutations in the genes, VSX1 (visual system homebox 1), TCF8, and CO8A2 seem to have genetic heterogeneity (Schmedt et al. 2012).
5.1.4 The Limbus
Between the cornea and the sclera is the limbus, a zone of approximately 1 mm that surrounds the cornea and is the site for surgical incisions for cataract and glaucoma. In the limbus, the stem cells for the epithelium as well as vascular elements reside in providing nutrients to the avascular cornea. The vasculature of the limbus derives in primates primarily from the anterior ciliary arteries (Van Buskirk 1989). The limbus has two important functions in maintaining a healthy corneal epithelium. It is the source of stem cells for the corneal epithelium; it also acts as a barrier separating the clear avascular corneal epithelium from the surrounding vascular conjunctival tissue. This microenvironment can be altered due to disease leading to limbal stem cell deficiency, when the corneal surface becomes replaced by hazy conjunctival tissue. The causes of limbal stem cell deficiency include, chemical injuries to the eye, inflammatory diseases, and hereditary diseases (Ahmad 2012).
5.1.5 Basement Membrane
Basement membranes are specialized acellular matrix that not only plays a role in separating the cellular layers but also connect them through the molecular matrix. Corneal epithelial basement membrane modulates the epithelial-to-stroma and stroma-to-epithelial interactions by regulating cytokines and growth factor movement from one cell layer to the other. In cornea, the basement membrane is present between epithelial cells and stroma. After 8 weeks of gestation, the epithelium is separated out from stroma by basement membrane which is avascular. The lamina lucida and lamina densa are the adjacent layers. The basement membrane is chiefly composed of the laminin, apart from collagen, heparan sulfate proteoglycans (HSPG), and nidogens. The composition varies from the limbal region to the central cornea. Laminins are heterotrimeric glycoproteins that are most abundant with different isoforms playing distinct roles. The gene defect has been associated with corneal diseases such as keratoconus, Fuchs dystrophy, and bullous keratopathy. Fibronectin, thrombospondin, matrilin, tenascin C, and nidogens 1 and 2 are the other major basement membrane matrix proteins that act as link proteins to laminin. The anchored proteoglycans such as perlecan, a complex multi-domain HSPG, can bind to various but discrete molecules. It regulates the availability of fibroblast growth factors (FGF), bone morphogenic proteins (BMP), platelet-derived growth factor (PDGF), vascular endothelial growth factors (VEGF), transforming growth factor β-1 (TGF-β1), and insulin-like growth factors (IGF) to bind to their receptors thereby mediates the cell signaling for migration, proliferation, and differentiation of a variety of cells. Perlecan is crucial for corneal epithelium formation and differentiation. Perlecan-deficient mice show epithelial defects. Mutations are reported in integrin, keratins, collagen subtypes, laminin, and many of the basement membrane proteins (Alarcon et al. 2009; Torricelli et al. 2013).
The hemidesmosome (HD) link is a complex of extracellular transmembrane and cytoplasmic molecules that form structural link between the intracellular keratin intermediate filament of cytoskeleton of the basal epithelial cell and the underlying basement membrane. The basement membrane zone has the hemidesmosome and the lamina lucida and lamina densa, which is the uppermost region of the stroma (Borradori and Sonnenberg 1999). Basement membrane normally functions as a barrier to the penetration of epithelial TGF-β1 and PDGF into the stroma. Loss of basement membrane leads to penetration of these factors that promote myofibroblasts development. Thus, basement membrane functions as the regulating barrier, limiting the fibrotic responses in injury, by regulating molecular interaction between the epithelium and the stroma. It plays a role in maintaining homeostasis of the matrix by downregulating wound healing signaling, modulation of epithelial cell growth proliferation and differentiation (Singh et al. 2011). Basement membrane also acts as a barrier to the bacterial penetration (Alarcon et al. 2009).
5.1.6 Descemet’s Membrane
Descemet’s membrane is a thick basement membrane (5–10 μm). Descemet’s membrane like other basement membranes consists of two distinct layers, a posterior layer adjacent to the endothelium and an anterior layer formed by collagen lamellae and proteoglycans (Kabosova et al. 2007).
5.1.7 Innervations in Cornea
An important characteristic of the cornea is its heavy innervations, with around 300 times the number of nerve endings per square mm of the skin. These are sensory nerves derived from the ciliary nerves of the trigeminal ganglion ophthalmic branch. The nerves penetrate the cornea through the peripheral stroma in a radial pattern and shed their myelin sheet, surrounded only by Schwann cell sheaths. They subdivide several times into smaller side branch and then advance to the epithelium to form the basal epithelial nerve plexes. The absence of a myelin sheath on central corneal axons is necessary to maintain corneal transparency. Corneal nerves exert a trophic effect on the epithelium and maintain the health of corneal epithelium. Dysfunction of the nerve causes degenerative neurotrophic keratitis resulting in decreased mitotic activity of the basal epithelial cells, decreased epithelial thickness, increased epithelial permeability, loss of cellular glycogen stores, decreased oxygen uptake rates, and altered hypoxic swelling responses. Table 5.1 shows the various trophic factors released by the corneal nerve endings and their response (Muller et al. 2003).
Table 5.1
Neuropeptides and nerve growth factors in the cornea
S. No. | Name of the factor | Effect/acts on | Reference |
---|---|---|---|
1 | Substance P | Stimulation of corneal epithelial cell proliferation | Garcia-Hirschfeld et al. (1994) |
Migration | Nishida et al. (1996) | ||
Adhesion | |||
2 | Calcitonin gene-related peptide | Colocalizes with SP in ocular nerve fibers; epithelial renewal and wound repair | Jones and Marfurt (1991, 1998), Muller and Klooster (2001) Mertaniemi et al. (1995) |
3 | Norepinephrine | Stimulates corneal epithelial cell proliferation and migration | Voaden (1971), Murphy et al. (1998) |
Some other studies suggest that adrenergic nerves exert inhibitory influences on epithelial cell proliferation and migration | Friedenwald and Buschke (1944), Reidy et al. (1994) | ||
Catecholamines | Stimulate epithelial chloride ion transport from stroma to tears in rabbit cornea | Klyce and Crosson (1985) | |
4 | Acetylcholine | Stimulates epithelial cell DNA synthesis | Cavanagh and Colley (1982), Colley et al. (1985) |
Increases cyclic GMP production | Cavanagh and Colley (1982) Walkenbach and Ye (1991) | ||
Activates the phosphoinositide cycle | Proia et al. (1986) | ||
Cholinergic stimulation of keratinocytes, promotes cell-to-cell cell-to-substrate adhesion and cell motility | Grando et al. (1995), Zia et al. (1997) | ||
5 | Vasoactive intestinal polypeptide | Stimulates corneal epithelial cell production of nerve growth factor | Campbell et al. (2001) |
6 | Neurotensin | Increases keratocyte proliferation and viability and decreases keratocyte apoptosis in cultured human corneal keratocytes | Bourcier et al. (2002) |
7 | Met-enkephalin | An inhibitor of wound healing | Zagon et al. (1998) |
8 | Neurotrophin 3 (NT-3) | Binds with TrkB and initiates signal transduction cascade leading to gene transcription | Patapoutian et al. (1999), Dechant and Barde (2002) |
Neurotrophin 4/5 (NT-4) | |||
Brain-derived neurotrophic factor (BDNF) | |||
9 | Nerve growth factor | Homeostasis and regeneration of epithelium and stroma in human cornea | |
Anti-inflammatory and healing promoting in murine immune corneal ulcers with stromal melting | Bonini et al. (2000) | ||
Enhances migration of corneal epithelial cells for wound healing | Murphy et al. (2001) | ||
Regulate corneal epithelial stem cells | Schermer et al. (1986), Touhami et al. (2002) | ||
10 | Ciliary neurotrophic factor | Released in response to oxidative stress by corneal Endothelial cells via VIP synthesis in sympathetic ganglia | |
Neurite outgrowth of peripheral sensory nerves | White et al. (2000) | ||
11 | Glial cell-derived neurotrophic factor | Synthesized by keratocytes in stroma to modulate the function of corneal epithelial cells | You et al. (2001), Ebner et al. (2001) |
Migration of corneal epithelial cells in Boyden chambers and in an in vitro wound healing assay and induced MAP-kinase signaling |
5.1.8 Innate Immunity of the Cornea
Though the cornea is avascular, during injury there is an inflammatory response characterized by stromal cell infiltration of polymorphonuclear neutrophils (PMNs) that come from the limbal vessels followed by a cascade of events involving anti-inflammatory mediators. If inflammation is not resolved, then corneal fibrosis, pigmentation, and neovascularization take place, resulting in corneal scarring and disruption of the blood ocular barrier, resulting in chronic immune-mediated uveitis (Grahn and Cullen 2000).
Ocular immune system protects the eye from infection and regulates healing processes following injuries. The eye is an immune-privileged site and is endowed with remarkable properties that permit the long-term survival of foreign tumor and tissue grafts due to multiple anatomical, physiological, and immunoregulatory processes. Innate immune responses provide an inherent barrier against corneal infection while also serving as a primary mode of defense that is present from birth. The most evident is the tear film that coats the cornea and conjunctiva and serves several important functions such as lubrication, supply of oxygen, and protection against a range of potential pathogens. Ocular tissues and fluids express a wide variety of anti-inflammatory and immunosuppressive molecules, including lysozyme, lactoferrin, lipocalin, calcitonin gene-related peptide, somatostatin, and complement regulatory proteins. Table 5.2 lists the various antimicrobial proteins and peptides in the tear.
Table 5.2
Antimicrobial proteins and peptides in tear
Protein/peptide | Composition | Effective against | Mode of action | References |
---|---|---|---|---|
Lysozyme | 20–30 % | Gram-positive bacteria | Hydrolysis of 1,4-beta-linkages between N-acetylmuramic acid and N-acetyl-d-glucosamine in the peptidoglycan | Sack et al. (2001) Aho et al. (1996) Albert (2008) |
Fungi | Cleaves chitodextrins in fungal cell wall | Lee-Huang et al. (1999) | ||
Lactoferrin | 20–30 % | Bacteria, fungi, and viruses | Binds divalent cations including iron depriving the bacteria of essential growth nutrient Acts as cationic detergent and disrupts the cell membrane of some bacteria, fungi, and virus | Janssen and van Bijsterveld (1983) Sack et al. (2001) Farnaud and Evans (2003) |
Lipocalin | 25 % reflex tear | Bacteria and fungi | Binds to microbial siderophores Protects ocular surface from microbial cysteine proteases | Dartt (2011) |
Secretory Immunoglobulin A | Major Ig in tear | Neutralize pathogen preventing their host attachment Binds to lectin-like adhesion molecule of pathogens Chemotactic to phagocytic neutrophils | ||
Complement fragments | Low levels in basal tear. Not present in reflex tear | Alternate complement pathway activation leading to formation of membrane attack complex | Willcox et al. (1997) | |
Cytokeratin 6A | Bacteria | Induce cell wall/membrane permeabilization, inhibit bacterial motility | Tam et al. (2012) | |
Secretory phospholipase A (sPLA2) | Major tear protein | Gram-positive bacteria | Binds to the anionic bacterial surface, kills via its lipolytic enzymatic activity. hydrolyzes the sn-2-fatty acyl moiety from phospholipids | Buckland et al. (2000) Nevalainen et al. (2008) |
Secretory leukocyte protease inhibitor (SLPI) | Reflex tears, higher amount in closed eye tears | Active against both Gram-positive and negative bacteria, fungi, and HIV | High cationic charge, binds anionic bacterial surface, inhibits neutrophil elastase | Franken et al. (1989) Sallenave (2010), Sathe et al. (1998) |
Elafin | Low concentration | Bacteria | Directly antimicrobial | Sallenave (2010) |
Bactericidal/permeability-increasing protein (BPI) | Low concentration | Gram-negative bacteria | Bind LPS and kill | Peuravuori et al. (2006) Holweg et al. (2011) |
β-lysin | Gram-positive bacteria | Targets the bacterial cell membrane. Inhibits bacterial catalase and peroxidase | Ford et al. (1976) | |
Surfactant protein (SP) A and D [members of C-type collectins) | Gram-negative bacteria | Bind pathogens and regulate host defense, block microbial traversal in the cornea | ||
Antimicrobial peptides (AMPs) (<50 amino acids) | Bacteria | Disruption of microbial cell membranes leading to cell death | Choi et al. (2012) | |
α-Defensins, β- defensins | Basal and reflex tears | Direct antimicrobial activity | ||
Glycoproteins | ||||
MUC1, MUC4, MUC16 Gel-forming MUC5Ac | Trap pathogens helping to move them to the lacrimal drainage pathway, facilitating their removal from the surface |
Toll-like receptors present at the ocular surface render additional immune barrier by triggering the earliest immune response to the invading pathogens. The TLRs act by modulating the adaptive immune response which is mediated by MyD88 (myeloid differentiation factor 88). Additionally, certain TLRs can also induce TRIF {TIR [Toll/IL (interleukin)-1 receptor] domain-containing adaptor protein which induces IFN (interferon) β}-dependent signaling (Kenny and O’Neill 2008). The activation of these intracellular signaling pathways induces the expression of cytokines, chemokines, and adhesion molecules.
Around, 10 TLRs (TLR1–TLR10) have been shown to be present in humans (Akira et al. 2001; Yu and Hazlett 2006). Different TLRs play a key role in the regulation of the response to infectious agents in the cornea: TLR4 and TLR5 are involved in Gram-negative; TLR2 in Gram-positive bacterial infections, TLR2 and TLR4 are activated in fungal keratitis; TLR3, TLR7, and TLR8 are involved in viral infections. Both the bacterial and viral genomes are able to activate a TLR9 response. Lambiase et al. have reviewed the scope of toll-like receptors at ocular surface (Lambiase et al. 2011).
Reports suggest that TLRs may become therapeutic targets in inflammatory ocular diseases, associated with the possibility to use extracellular TLR agonists. Pharmacological modulation of TLR intracellular signaling pathways may allow novel therapeutic strategies for avoiding the detrimental effects of prolonged inflammation at the ocular surface. The evaluation of TLR-modulating molecules in human diseases has already started with several ongoing clinical trials (Hennessy et al. 2010). There is a rapidly growing literature on TLRs in the cornea and conjunctiva, opening the possibility of targeting these molecules in the management of ocular surface diseases.
5.1.9 Growth Factors in Corneal Wound Healing
5.1.9.1 Epithelial Growth Factor (EGF)
It is a family of 13 growth factors that includes EGF, TGF-α, and heparin binding EGF (HB-EGF). The receptor, namely, EGFR, acts as a cell surface receptor. Four related receptor tyrosine kinases include EGFR/erbB1/HER1, erbB2/HER2, erbB3/HER3, and erbB4/HER4 (Schultz et al. 1991), all of which have been detected in the corneal epithelium. EGF is secreted by platelets, macrophages, and fibroblasts and act on epithelial cells (Hynes et al. 2001). Heterodimerization of EGF receptor tyrosine kinases results in the activation of the signaling cascade resulting in corneal epithelial migration, thereby promoting epithelial wound healing (Fig. 5.3).
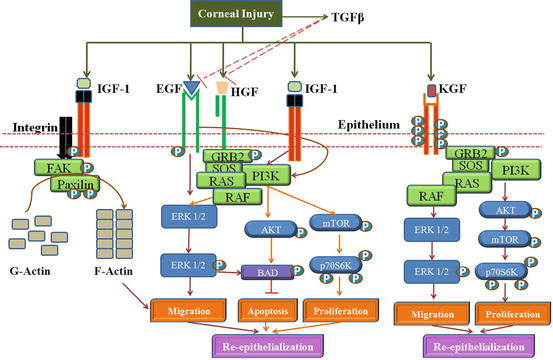
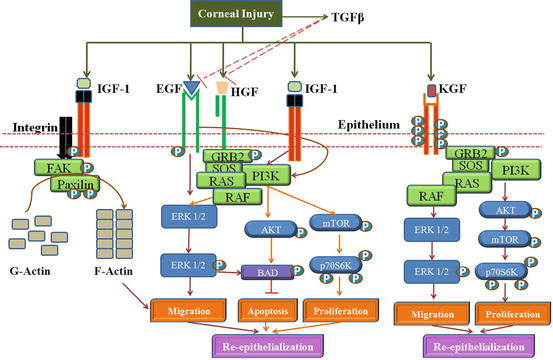
Fig. 5.3
Interplay of Growth factors in Corneal Wound healing: The growth factors, EGF, HGF, KGF and IGF-1 promotes cell proliferation through the activation of the Pi3K-AKT pathway. EGF enhances migration through the ERK1/2 pathway. IGF-1 and Substance-P (S-P) synergistically activate integrin-5 mediated actin polymerization via Fak-Paxilin t promote migration of the epithelial cells. KGF in limbal epithelium promotes migration and proliferation through the ERK1/2 and PI3K pathway. TGFβ secreted by epithelium can prevent re-epithelization by inhibiting EGF and HGF signaling
5.1.9.2 Keratinocyte Growth Factor (KGF)
Keratinocyte growth factor (28 kDa), a fibroblast growth factor known as FGF-7, is a potent mitogen for the corneal epithelial cells. It is produced by the conjunctival and the stromal fibroblasts (Wilson et al. 1999). However, KGF exerts paracrine effect on the corneal and the limbal epithelial cells, increases cell proliferation, thereby promoting wound healing effect. KGF activates Ras-MAPK and PI3K/p70 S6 pathways in the corneal epithelial cells and the p38 pathway in the limbal epithelial cells (Chandrasekher et al. 2001; Cheng et al. 2009).
5.1.9.3 Hepatocyte Growth Factor (HGF)
Hepatocyte growth factor (69 kDa of α-chain and a 34 kDa of β-chain) produced by mesenchymal cells stimulates the tyrosine activity of high-affinity receptor, c-Met, a proto-oncogene product expressed in epithelial cells (Bottaro et al. 1991). HGF is produced mainly by fibroblasts and has a paracrine action on the epithelium. While KGF is produced in the limbal cornea, HGF is produced more centrally. HGF-facilitates corneal epithelial cell migration and proliferation and inhibits apoptosis (Yu et al. 2010). HGF activates Ras-MAPK pathways via the receptor-Grb2/Sos complex (Liang et al. 1998). HGF is also reported to transactivate EGFR (Spix et al. 2007).
5.1.9.4 Insulin-like Growth Factor-1 (IGF-1)
Insulin-like growth factor-1 and its receptors are expressed by both epithelial cells and fibroblasts of the cornea (Li and Tseng 1995). IGF has been shown to induce all migration through activation of PI3K/AKT pathway, while in corneal epithelial cell it enhances proliferation and inhibits apoptosis (Lee et al. 2006). IGF-1 increases chemotaxis in the corneal fibroblasts (Yanai et al. 2006). IGF seems to have a synergistic effect with substance P in corneal wound healing (Nakamura et al. 1997) and upregulates FAK, paxilllin, and integrin alpha 5 (Nakamura et al. 1998a, b). IGF also a plays a role in cell communication via gap junctions through connexin 43 expression in fibroblasts (Ko et al. 2009). Insulin is present in human tear film, and its receptors are seen in the corneal epithelial, keratocytes, and conjunctival cells. Systemic and topical application of insulin promotes re-epithelialization in the cornea.
5.1.9.5 Transforming Growth Factor-β (TGF-β)
TGF-β family has three members, TGF-β1, TGF-β2, and TGF-β3. They regulate cell proliferation, extracellular matrix synthesis, angiogenesis, immune response, apoptosis, and differentiation (Andresen and Ehlers 1998; Roberts 2002). Latent TGF-β binding proteins (LTBPs) regulate TGF-β bioavailability, activity, and secretion. TGF-β 1 and TGF-β 2 are seen in the corneal epithelium and stroma and tear fluid with higher levels of TGF-β2. The TGF-β receptors RI and RII are located in the epithelial, stromal, and endothelial layers of the cornea (Andresen et al. 1997; Nishida et al. 1994). While TGF-β1 and TGF-β 2 inhibit corneal, endothelial cell proliferation, they stimulate stromal fibroblast proliferation (Yanai et al. 2006; Kay et al. 1998). TGF-β1 delays re-epithelialization, increases keratocyte proliferation, and promotes myofibroblast differentiation. It increases the connective tissue growth factor (CTGF) levels and ends up in increased collagen synthesis and scar formation as seen in the corneal fibroblasts (Folger et al. 2001). Corneal fibrosis and haze in Photorefractive Keratectomy (PRK) is inhibited by TGF- β inhibition as shown in rabbits (Jester et al. 1997).
Corneal epithelial cell proliferation promoted by KGF and HGF is inhibited by TGF-beta, while in keratocytes it promotes the growth-promoting effect of EGF, thus functioning based on other growth factors in the environment. EGFR is transactivated by GPCR (G protein-coupled receptor), a central mediator that converges multiple extracellular signals in corneal epithelial wound healing. The two major signaling pathways are ERK and PI3K/AKT. Non-EGF family growth factors, namely, insulin, IGF, and HGF, also activate ERK and PI3K pathways in endothelial cells (Hongo et al. 1992; Honma et al. 1997).
Chemical signals in the injury process are the cellular proteins now termed as “alarmins,” that include high mobility group box 1 and heat shock proteins, antimicrobial peptides at the site of injury as a defense to inhibit further damage by release of lipid autocoids, lysophosphatidic acid, adenosine, and other nucleotides which can further act as alarmins (Yu et al. 2010). Many purinoreceptors are expressed by the corneal epithelial cells. Release of high levels of ATP by the injured cells can activate the P2Y receptors that results in EGFR transactivation via GPCR activation and calcium wave signaling that in turn promotes wound healing (Yin et al. 2007).
5.1.10 Lipids in Cornea
The neutral lipids form the major composition in human cornea, which is 34 %. This includes cholesterol, cholesterol esters, and triglycerides followed by the sphingolipids (24 %) and the gangliosides (10 %) (Feldman 1967). The phospholipids in cornea include phosphatidyl choline, phosphatidyl ethanolamine, and sphingomyelins (Rodrigues et al. 1983). The fatty acids predominantly seen are oleic acid, palmitic acid, and stearic acid (Tschetter 1966).
The tear film lipid layer consists of a mixture of nonpolar lipids (wax esters, cholesterol, and cholesterol esters), which is 60–70 % of the layer; the rest is composed of phospholipids, glycolipids, and a small amount of free fatty acids, including omega fatty acids and mono- and diglycerides (Andrews 1970; Tiffany 1987). Tear lipids arrive from meibomian glands and the accessory sebaceous gland of Zeis. The phospholipids secreted by the meibomian gland act as amphiphilic molecules to form an interphase between the outer nonpolar lipid layer and the inner aqueous layer (Pucker and Haworth 2015). Dietary intake of omega fatty acids and its topical application is shown to be effective against dry eye (Roncone et al. 2010).
Corneal arcus results from lipid infiltration into the peripheral cornea in humans. These lipids are cholesterol-rich lipid particles deficient in apolipoprotein (apo)-B (Cogan and Kuwabara 1959). Lipid keratopathy is another condition common in women, often associated with hypercholesterolemia with cholesterol esters as droplets deposited in the stroma by lipid-overloaded fibroblasts (Gaynor et al. 1996). Corneal neovascularization can pave way for lipid access to the cornea; herpetic keratitis and chemical injuries produce severe lipid keratopathy (Forsius 1961). Another disease, granular corneal dystrophy, an autosomal dominant heritable condition, is characterized by granular deposits in the cornea and shows an increase in phospholipids with no changes in cholesterol (Rodrigues et al. 1983). Lipids are known to be involved in the pathology of dry eye with the loss of the lipid layer thickness being associated with the severity of the dry eye (Foulks 2007).
Injury to the cornea stimulates the release of platelet activation factor (PAF), a bioactive lipid that activates phospholipoase A2 (PLA2). This results in the release of arachidonic acid (AA) as well as its conversion to prostaglandins (PGD), thromboxanes (TX), and lipoxygenase (LOX) derivatives (Bazan 1987; Bazan et al. 1985a, b) in all the three layers of the cornea. Cycloxygenase-1 (COX-1) is expressed throughout the cornea, whereas COX-2 is predominantly expressed close to the wound (Amico et al. 2004). Laser in situ keratomileusis (LASIK), which damages the stroma, induces expression of COX-2 in the central epithelium and in the keratocytes adjacent to the wound (Miyamoto et al. 2004). COX-2 metabolites contribute to tissue damage and neovascularization. LOX derivatives from arachidonic acid, 12- and 15-hydroxy/hydroperoxy eicosatetraenoic acids, and lipoxin A4 resolvins (RvE1) from eicosapentaenoic acid (EPA) and neuroprotectin D1 (NPD1) from docosahexaenoic acid (DHA) potentiate repair and regeneration. Stimulation of the cornea with pigment epithelial-derived factor in the presence of DHA gives rise to the synthesis of NPD1, a derivative of LOX activity, and increases regeneration of corneal nerves (Kenchegowda and Bazan 2010).
15-hydroxy/hydroperoxyeicosatetraenoic acid [15(S)-HETE] formed from arachidonic acid (AA) by LOX action has a protecting effect from the dry eye (Gamache et al. 2002) and promotes mucin secretion (Jackson et al. 2001). While LOX derivatives are protective agents in the cornea, PAF plays a central role in mediating corneal inflammation, cell death, and neovascularization.
Corneal injury causes altered levels of growth factors that activate 12/15-LOX. Prostaglandins show a variety of effects in cornea through the eicosanoids (Table 5.3). A balance of pro- and anti-inflammatory levels of bioactive lipids decides the fate of corneal injury.
Table 5.3
Lipid mediators in corneal injury
Lipid | Source/site of action | Mode of action | References |
---|---|---|---|
Eicosanoids: PGD2 | Conjunctival epithelium | Causes epithelial defects though chemotaxis of eosinophils in the conjunctiva. | Fujishima et al. (2005) |
PGE2 | Corneal endothelium Myofibroblasts | Inhibit endothelial cell proliferation. Causes corneal nerve stimulation. | |
Prostaglandins (PG): Cytosolic PLA2 (cPLA2) α and γ; secretory PLA2s (sPLA): GIII, GX, and GXIIIA | Seen in the corneal epithelium and tears PLA2 activation occurs in the epithelium after stimulation by platelet-activated factor (PAF) | Provides antibacterial protection after corneal injury. cPLA2α releases. In corneal injury that increases COX and LOX metabolites. PAF, a bioactive lipid that activates PLA2 increases after corneal injury. Activators of MMPs in the cornea and conjunctiva. | Landreville et al. (2004) Hurst and Bazan (1995) Bazan et al. (1991) Ottino and Bazan (2001) |
Oxidized lipids HETEs: 15(S)-HETE: a product of 15-lipoxygenase (LOX) Other oxidized lipids: leukotrienes, lipoxins, and hepoxilins Lipoxin A4 (LxA4), another product of 12/15-LOX | Corneal epithelium Human and monkey corneas express 15-LOX-1 in the cytoplasm and 15-LOX-2 in the cytoplasm and nucleus of mouse/rabbit corneas ALX in human corneal endothelial cells | HETE brings about PKC alpha translocation to plasma membrane via MAPK/ERK and cPlA2 when stimulated by EGF. HGF. 12(S)- and 15(S)-HETEs act as lipid mediators in the cornea for the proliferation. EGF released after corneal injury increases epithelial proliferation through induction of 12/15-LOX. Stimulate epithelial wound healing LxA4 synthesis is stimulated by EGF. LxA4 and epi-LxA4 act through a G protein-coupled receptor (ALX/FPR2). | Sharma et al. (2005) Gronert et al. (2005) Kenchegowda et al. (2009), He et al. (2008) |
Cytochrome P450 monooxygenase: two main compounds are formed: 12-R-hydroxyeicosatetraenoic acid [12(R)-HETE], and its degraded product, 12-R-hydroxyeicosatrienoic acid [12(R)-HETrE] | Cornea and tear | 12(R)- HETrE is a potent inflammatory and angiogenic mediator in hypoxia (contact lens wear/chemical injuries) in the cornea. Increases in human tears during inflammation. These compounds diffuse across the stroma and inhibit the endothelial Na+/K+ -ATPase. | |
Platelet activation factor (PAF) | All corneal cells except fibroblasts. It is increased by growth factors that are upregulated in injury: KGF, TGF-beta, and HGF | Inflammatory mediator through PAF-R receptor coupled to G proteins. Inhibits epithelial wound healing PAF activates MMP-1, MMP-9, and uPA PAF attracts PMNs and other leukocytes to the site of injury PAF activates MAPK-ERK1/2 PAF also triggers the Ca2+ influx in corneal epithelial cells that could also be involved in cPLA2 activation PAF induces stromal keratocyte apoptosis by activation of NF-κB | He et al. (2006) |
ω-3 Fatty acids and their derivatives α-Linolenic acid and to the elongation and desaturation products: eicosapentaenoic acid (20:5, EPA) and docosahexaenoic acid (22:6, DHA) Derivatives of EPA: 5,12,18-R-trihydroxy-eicosapentaenoic acid called resolvin E1 (RvE1) DHA derivative: neuroprotectin D1 (NPD1: 10R, 17S-dihydroxy-docosa-4Z,7Z,11E,13E,15Z,19Z- hexaenoic acid) | Animal models: corneal inflammation Dry eye In the cornea, DHA is a minor component of membrane phospholipids In the cornea, neurotrophins as well as the corresponding Trk-receptors are expressed in epithelial cells and keratocytes | Blocks PMN infiltration and reduces leukocyte-mediated tissue injury Increases tear production as well as epithelial cell density Reduces corneal neovascularization by reducing infiltrated neutrophils/macrophages and the gene expression of inflammatory cytokines A role in regenerating corneal innervation and has a synergistic effect with PEDF Promotes epithelial cell proliferation | Serhan et al. (2008) Li et al. (2008), ARVO Cortina et al. (2010) |
5.1.11 The Lacrimal Functional Unit and the Tear Film Dynamics
The tear film is considered a vital structure whose main role is to protect the ocular surface from external environment, infectious agents, and desiccation. The multiple functions of tear fluids include: lubrication of the eyelids, formation of a smooth and even layer over the cornea and conjunctiva, providing antibacterial systems for the ocular surface and nutrients for the epithelium, act as a vehicle for the entry of polymorphonuclear leukocytes in case of injury, and washing away the toxic irritants (Lemp and Blackman 1981; Braun et al. 2015).
Tear film is a trilaminar structure consisting of: (1) a thin anterior lipid layer (0.1 μ) originating from the meibomian glands which contains both polar and nonpolar lipids; (2) an intermediate aqueous layer (7 μ) originating from the lacrimal glands, which contains water soluble substances, including electrolytes, proteins, retinol, immunoglobulins, and enzymes- and (3) innermost mucous layer (0.02–0.04 μ) – secreted by conjunctival goblet cells and lacrimal gland acinar cells and containing components loosely bound to glycocalyx of the corneal and conjunctival epithelial cells.
Tear is composed of 98 % water and 2 % solids, like proteins, lipids, carbohydrates, and salts. The total tear volume produced is around 6.2 μl, ranging from 3.4 to 10.7 μl. The thickness of tear film is 6–7 μm; the pH slightly basic, i.e., 7.5; and the osmolarity is 300–334 milli osmolar (Jordan and Baum 1980). Sodium, potassium, chloride, bicarbonate, magnesium, and calcium salts are found in the tear. The chloride concentration is more in tears compared to the serum (Van Haeringen 1981). Tear has metabolites such as glucose, urea, lactate, pyruvate, ascorbate, retinol, free amino acids, uric acid and bilirubin.
The tear film is thus a complex mixture of proteins, lipids, mucus, salts, enzymes, and other metabolites produced from multiple sources such as the lacrimal gland, meibomian gland, goblet cells, and accessory lacrimal glands of the ocular surface. Glands of Krause located in the lamina propria of the conjunctival fornices (superior and inferior), Moll glands at the base of the lashes anterior to the meibomian glands and Wolfring located above the superior border of the upper lid tarsus contributes to the secretion of components into the lacrimal fluid (Lamberts et al. 1994). The lacrimal gland is a paired, almond-shaped gland, one for each eye that secretes the aqueous layer of the tear film. They are situated in the upper, outer portion of each orbit, in the lacrimal fossa of the orbit formed by the frontal bone (Walcott 1998). The lacrimal glands secrete lacrimal fluid as a result of neuronal stimuli, which flows through the main excretory ducts into the space between the eyeball and lids and during blinking of eyes. The lacrimal fluid is spread across the surface of the eye accumulating in the lacrimal lake and is drawn into the puncta by capillary action, flowing through the lacrimal canaliculi to the inner corner of the eyelids entering the lacrimal sac, then to the nasolacrimal duct, and then finally into the nasal cavity. Lacrimal functional unit (LFU) is an integrated system including lacrimal gland, ocular surface, lids, and sensory and motor nerves, to carry out its function. The lacrimal glands consist of a tubular secretory epithelium organized into lobes that drain into ducts. It is a multilobular tissue composed of acinar, ductal, and myoepithelial cells. The acinar cells account for 80 % of the cells present in the lacrimal gland and are the sites for synthesis, storage, and secretion of proteins. Several tear proteins like lysozyme, lactoferrin, growth factors such as epidermal growth factor and transforming growth factor are crucial to the homeostasis of the ocular surface.
Ductal cells: These cells modify the primary fluid secreted by the acinar cells to secrete water and electrolytes. Myoepithelial cells contain multiple processes, which surround the basal area of the acinar and ductal cells. They contain a smooth muscle actin that helps to contract and force the fluid out of the ducts and onto the ocular surface similar to salivary and mammary glands. Other cell types present in the lacrimal gland includes plasma cells, B and T cells, dendritic cells, macrophages, bone marrow-derived monocytes, and mast cells. Immunoglobulin A (IgA)-positive plasma cells are the majority of the mononuclear cells in the lacrimal gland (Dua et al. 1994; Wieczorek et al. 1988). These cells synthesize and secrete IgA, which is then transported into acinar and ductal cells and are secreted by these epithelial cells as secretory IgA.
5.1.11.1 Neural Control of Tear Secretion
The lacrimal gland is innervated by the parasympathetic and sympathetic nervous system (Botelho et al. 1966; Sibony et al. 1988). Nerves are located in close proximity to acinar, ductal, and myoepithelial cells as well as blood vessels, and they control a wide variety of lacrimal gland functions (Botelho et al. 1966; Sibony et al. 1988). Stimulation of lacrimal gland secretion occurs through a neural reflex arc originating from the ocular surface (Botelho 1964). Stimuli to the ocular surface activate afferent sensory nerves in the cornea and conjunctiva which in turn activates efferent parasympathetic and sympathetic nerves in the lacrimal gland to stimulate secretion.
Neurotransmitters and neuropeptides released by the lacrimal gland nerves include acetylcholine, vasoactive intestinal peptide (VIP), norepinephrine, neuropeptide Y (NPY), substance P (SP), and calcitonin gene-related peptide (CGRP). These neuromediators interact with specific receptors present on the surface of lacrimal gland cells to initiate a specific response (Hodges and Dartt 2003). Acetylcholine and norepinephrine are the most potent stimuli of for the secretion of lacrimal gland proteins, water, and electrolytes (Dartt 2004; Hodges and Dartt 2003). Acetylcholine binds to cholinergic M3 muscarinic receptors, and norepinephrine binds to α- and β-adrenergic receptors (Hodges and Dartt 2003; Mauduit et al. 1993) leading to respective stimulation.
5.1.11.2 Hormonal Control of Tear Secretion
Hormones from the hypothalamic-pituitary-gonadal axis has a profound impact on lacrimal gland structure and function. Adrenocorticotropic hormone (ACTH), alpha-melanocyte-stimulating hormone (a-MSH), prolactin, androgens, estrogens, and progestins influence lacrimal gland functions (Leiba et al. 1990; Mircheff et al. 1992; Sullivan et al. 1996). In addition, glucocorticoids, retinoic acid, insulin, and glucagon affect various aspects of the lacrimal gland (Petersen 1976; Rocha et al. 2000; Ubels et al. 1994). Arginine vasopressin, a peptide produced in the posterior pituitary, has physiologic role in fluid homeostasis, is present in the lacrimal gland acinar and ductal cells (Djeridane 1994). Androgens are potent hormones that stimulate the secretion of secretory IgA, an important component of the mucosal immune system of the eye (Sullivan et al. 1996). Androgens accounts for many of the gender-related differences seen in the lacrimal gland (Azzarolo et al. 1997; Sullivan et al. 1999).
Protein and water secretion by lacrimal gland (Walcott 1998): A number of proteins is synthesized and secreted by the lacrimal gland acinar cells. The secretion of these proteins is stimulated by the neurotransmitters and neuropeptides found in the neurons that innervate the gland (Dartt 2004). The acinar cells have receptors for acetylcholine (muscarinic M3), VIP, and norepinephrine and receptors for peptides of the proenkephalin family as well as other peptides such as neuropeptide Y, adrenocorticotropic hormone, and alpha-melanocyte-stimulating hormone. The acinar cells are extensively coupled by gap junctions. Second messengers produced by activation of these receptors, such as Ca2+ and inositol trisphosphate (IP3), diffuse from stimulated cells to adjacent non-stimulated ones, causing them to become activated, which further leads to protein secretion.
Water is the one of the major secretory products of the lacrimal gland. This water moves through the interstitial spaces of the gland into the lumen of the gland where it is mixed with the other secretory products. This water movement is accomplished by osmosis, which depends on the movement of ions from the acinar cells into the lumen (Dartt 2009). The acinar cell surface membrane is differentiated into basolateral and apical domain, which are separated by the junctional complex. The apical domain contains water channels (aquaporin 5), which facilitates the movement of water across the epithelium. In addition, Cl− and K+ channels are present to allow the movement of solute across the epithelium. The basolateral membranes contain large number of Na+ pumps (Mircheff 1989), Na+/K+-ATPase, which actively move K+ into the cell and Na+ out of the cell, maintains the gradients. It is this gradient (more Na+ outside and K+ inside) that provides the motive force for the movement of ions and water across the epithelium. In addition, there are several coupled transport systems (porters) driven by the concentration gradients created by the Na+ pump and by the activity of carbonic anhydrase. The basolateral membranes also have ion channels, specifically for K+, Cl−, and Ca2+ as well as more general cation and anion channels. This link between ion channels and their permeability and the movement of water may form the underlying mechanism of dry eyes.
5.1.11.3 Tear Proteins
Normal tears contain a total protein concentration of 6–10 mg/ml of tear and at least 80 tear proteins (Gachon et al. 1982) as reported. Major tear proteins include lysozyme, lactoferrin, secretory immunoglobulin A (sIg A), serum albumin (Li et al. 2005), lipocalin (Glasgow et al. 1995; Redl et al. 1992; Schoenwald et al. 1998), lipophilin (Lehrer et al. 1998) and tear-specific prealbumin (TSP), lacrimal proline-rich proteins (LPRR4) (Aluru et al. 2012), defensins, zinc-alpha-glycoprotein, mammaglogulins, and heat shock proteins originating from the lacrimal gland. Measured levels of proteins such as β2 microglobulin, cystatins, substance P, epidermal growth factor (EGF), transforming growth factor β1 and β2 (TGFβ1 and β2), plasmin, tryptase, and α1 antitrypsin change depending on the conditions. Other proteins include, mucins, antiproteinases, and bactericidal protein β-lysine (Ford et al. 1976). Growth factors, EGF, TGF-β1 and β2 are present in normal tear fluids and have been associated with corneal wound healing (Grus et al. 2001).
The glycoproteins in the tear includes orosomucoid, α1-glycoprotein, transferrin, ceruloplasmin, Zn-α2-glycoprotein, and mucous glycoproteins which contribute to the high viscous nature of the ocular mucus. The viscosity of human tears reduces during blinking, but between each blink it raises and thus stabilizes the tear film.
5.1.11.4 Tear Enzymes
A large number of enzymes including glucose-metabolizing enzymes, antiproteases, collagenases, matrixmetalloproteases, and other proteolytic enzymes are present in tears. During infections of the eye or in corneal ulcerations, the levels of some of these enzymes like α1-antitrypsin and α2-microglobulin are increased (Ford et al. 1976).
5.1.11.5 Tear Lipids
It includes free fatty acids, free sterols, triglycerides, diesters, polar lipids, and hydrocarbons. Meibomian secretion with its hydrophobic nature, functions as a barrier that prevents spill over of tears. The lipid layer reduces the rate of evaporation from an open eye, provides lubrication for the eyelid/ocular interface, and contributes to the optical properties of the tear film (McCulley and Shine 2001; McCulley and Shine 2003; Nicolaides et al. 1989).
5.1.12 Mucins in Tear
Glycoproteins are abundantly expressed, especially in the conjunctival cells and are abundantly found in tears. 424 glycogenes have been identified by the gene chip array of the conjunctival epithelium. Mucins are high-molecular-weight glycoproteins, and the carbohydrate-binding proteins form the major component of the tear glycoproteins (Mantelli and Argueso 2008). They have a characteristic property of tandem repeats of amino acids rich in serine and threonine to which O-glycan is attached (Gendler and Spicer 1995). Apical cell layers of the corneal epithelium produce mucin. MUC5AC is the major secreted mucin of the conjunctival goblet cells. The transmembrane mucins, MUC1, MUC4, and MUC16, are produced by the epithelium (Guzman-Aranguez et al. 2010).
Mucins along with galectin-3 promote barrier integrity and prevent bacterial adhesion. Galectin-3 is the most abundantly expressed carbohydrate-binding protein in human conjunctival epithelium, while galectin-8 and galectin-9 are found in much lower concentrations (Mantelli and Argueso 2008). Oligomerization of galectin-3 results in lattices that resist lateral movement of membrane components on the glycocalyx (Pablo Argüeso et al. 2013). Human tear mucins consist primarily of α2-6 sialyl core 1 (Galβ1-3GalNAcα1-Ser/Thr) (Guzman-Aranguez et al. 2009). However, in the conjunctiva, α2-3 sialyl core 1 is seen. The glycosylated mucins are hydrophilic and are responsible for the water-binding capacity that prevents ocular surface drying. In addition to mucins, glycosyltransferases, proteoglycans, glycan degradation proteins, and Notch signaling molecules are expressed on the ocular surface. They act as receptors for the pathogens, thereby trapping and removing pathogens from the epithelial surfaces. They prevent barrier function by preventing endocytosis (Guzman-Aranguez et al. 2012). Thus, lubrication and clearance of pathogens and debris are the major functions of the mucins and other glycoproteins in the tear.
5.2 Biochemistry of Lens
The lens of the vertebrate eye is a unique organ in that it is nonvascularized and noninnervated (Bloemendal 1981). The entire organ is composed of cells of surface ectodermal origin at various stages of differentiation surrounded by a basal lamina, the lens capsule (Duncan 1981; Bloemendal 1981). The epithelial cells ranging from 9 to 17 mm in diameter (Brown and Bron 1987) remain quiescent in the central epithelium (nondividing phase), surrounded by a germinative dividing zone of cells which divide toward the equatorial area (dividing phase) and terminally differentiate (differentiating phase) into long, transparent fiber cells in the equatorial region (Papaconstantinou 1967; Piatigorsky 1981). The transition of lens cortex into fiber cells during the developmental stages is characterized by biochemical changes which includes the synthesis of fiber-specific proteins, α- and β-crystallins, and arid morphologic changes such as cell elongation, loss of cellular organelles, and disintegration of the nucleus (Worgul et al. 1989). The single monolayer of anterior epithelial cells in the mature lens represents a very static population and is essential for maintaining the metabolic homeostasis and transparency of the entire lens (Spector et al. 1995). Anteriorly, they form an epithelial monolayer, while internally they are represented by shells of concentrically arranged fully differentiated fiber cells, which form the bulk of the lens (Winkler and Riley 1991). Thus, the lens continually grows throughout life and maintains its distinct polarity with the monolayer of epithelial cells covering only the anterior half of the fibers (McAvoy and Chamberlain 1989).
5.2.1 Glucose Metabolism in the Lens
The deeper fibers which make up most of the adult lens are organelle-free, while most of superficial fibers are metabolically active and are nucleated. Since the lens must be a transparent and colorless medium, the energy required for its growth and transparency is derived chiefly from glucose. To maintain transparency, the lens cannot be endowed with pigmented oxidative enzymes of the cytochrome system or the riboflavin-containing group. Thus, in the lens, glucose is catabolized primarily to lactic acid, and is not appreciably combusted to CO2 (Kinoshita et al. 1961). Anaerobic glycolysis yields about 70 % of the ATP (Winkler and Riley 1991). Aerobic incubation done in the absence of glucose indicates that the aerobic phase of metabolism is capable of providing energy to a certain degree. To support the energy-utilizing mechanisms, the Krebs cycle and the oxygen-utilizing mechanisms are sufficiently active to consume enough endogenous substrates (Piere and van Heyningen 1956). The ability of the lens to synthesize sorbitol is an unusual feature of lens glucose metabolism. The lens is rendered a favorite site for sorbitol production due to reasons like high pentose shunt activities, relatively high aldose reductase, and low hexokinase (Van Hayningen 1962).
5.2.2 Sorbitol Metabolism and Aldose Reductase
During normoglycemia, glucose entering the cell is rapidly phosphorylated by the enzyme hexokinase and enters the Embden-Meyerhof pathway, providing the major aerobic energy source for the cell (Kador et al. 1985). This pathway is fully saturated at normal glucose levels, and hence if hyperglycemia occurs, the excessive glucose cannot be metabolized by this pathway. The enzyme aldose reductase (AR) has a much lower affinity for glucose than hexokinase, and significant amounts of glucose enter the sorbitol (or polyol) pathway only when its levels are raised. AR converts glucose to the alcohol sorbitol, which is then further oxidized to fructose by the enzyme sorbitol dehydrogenase (Kador et al. 1985)
5.2.3 Transport of Fluid by Lens Epithelium
The lens is an avascular tissue which is thought to be nourished by diffusion. Yet, the metabolic consumption of nutrients by the lens cannot be attributed to simple diffusion of nutrients into the lens (Harris et al. 1955). Studies have shown lens fibre cells membranes to be rich in ion channels (K+, Na+, Ca2+), water channels (aquaporins), pumps (Na+/K+-ATPase) (Mathias and Rae 2004), and specific transporters for small molecules such as glucose and amino acids (Lim et al. 2006, 2007). Four mechanisms that govern the solute transfer from the aqueous and vitreous humors to the lens fibers in order of relevance are as follows:
(i)
Na+ -leak conductance drives the paracellular transport of water, small molecules, and ions along the intercellular spaces between epithelial and FCs.
(ii)
Specific carriers and transporters to bring about membrane transport of such solutes from the intercellular spaces to the fiber cytoplasm.
(iii)
Gap-junctional coupling mediating solute flux between superficial and deeper fibers, efflux of waste products in the equator, and electrical coupling of fibers driven by Na+/K+ -ATPase.
5.2.4 Lens Crystallins
The structural proteins abundantly seen in the lens that are evolutionarily related to stress proteins are the two crystallin gene families which comprise of the α-crystallins and the βγ-crystallins. α-Crystallins which possess chaperone-like activity in vitro are molecular chaperones that prevent aberrant protein interactions. Their activity includes remodeling and protecting the cytoskeleton, inhibition of apoptosis, and enhancement of the resistance of cells to stress. Binding to proapoptotic proteins such as p53 may be the possible mechanism of action for inhibition of apoptosis in lens (Jolly and Morimoto 2000); α -Crystallins are known to prevent undesired interactions and aggregation of cytoskeletal proteins (Horwitz 2003). Proteins of the βγ-crystallin family proteins are suggested to affect lens development, and its expression is seen in tissues outside the lens. Of the transcription factors regulating expression of different crystallin genes in lenses of different species, the most commonly studied are Pax-6, retinoic acid receptors, maf, Sox, CREB, and AP-1 (Cui et al. 2004; Cvekl and Piatigorsky 1996; Cvekl et al. 1994). Recent work demonstrates that targeted disruption of a DNA repair molecule, Nbs, affects transcription of crystallins (Yang et al. 2006).
5.2.5 Connexins in Lens
Connexins [Cx] are a family of membrane proteins that have two extracellular loops, one intracellular loop, four transmembrane domains and intracellular C as well as terminus N-terminus. In mammals, almost all cell types express one or more Cx isoforms. Cx43 is the most ubiquitous (Beyer et al. 1987; Dupont et al. 1988). Cx46 is present in lens (Paul et al. 1991) and lung (Abraham et al. 1999). Mammalian lens express three connexin isoforms. Cx43 and Cx50 is expressed by cortical epithelial cells (Dahm et al. 1999), whereas nuclear fiber cells express Cx46 and Cx50 (Tenbroek et al. 1992) and Cx50 (Kistler et al. 1985; White et al. 1992). Cx46 and Cx50 expressed by mature fiber cells are needed for the coupling of both peripheral and interior fiber cells (Baldo et al. 2001; Gong et al. 1998; Martinez-Wittinghan et al. 2004) and for maintaining lens transparency (Benedek 1971; Mathias et al. 1997; Hejtmancik 2008).
5.2.6 Aquaporins in Lens
The predominant membrane protein present in mature fibers is aquaporin 0 [AQP0], also called the major intrinsic protein [MIP]. AQP0 functions as a water channel at a pH of 6.5 and forms thin junctions of 11–13 nm thickness between the lens fibers at low calcium concentrations (Nemeth-Cahalan and Hall 2000); it also acts as an adhesion molecule interacting with Cx50 for the differentiation of lens fibers and to enhance gap junction formation (Engel et al. 2008; Kumari and Varadaraj 2009; Yu and Jiang 2004; Liu et al. 2011). Studies show that MIP (AQP0) expression in the adult bovine lens is seen in thin, asymmetric membrane interactions set apart from the thicker, symmetric junctions containing connexins (Gruijters et al. 1987).
5.2.7 Apoptosis in Lens Epithelial Cells
Programmed cell death or apoptosis is one of the features of the early steps in eye development associated with morphogenesis. Suppression of programmed cell death in the later stages is essential for tissue differentiation, and in the adult, factors inducing inflammatory cell apoptosis maintain the immune-privileged status of the eye (Lang 1997). Fibroblast growth factor is one of the components that acts during morphogenesis to bring about suppression of apoptosis in cells of the lens lineage (Bozanic et al. 2003). Human cataract patients have a significant percentage of apoptotic epithelial cells, while normal human lenses of similar age do not (Li et al. 1995a), and lens epithelial cell apoptosis can be activated by oxidative stress (Spector et al. 1995) and calcimycin (Li et al. 1995b) that cause cataract.
5.2.8 Epithelial to Mesenchymal Transition (EMT)
The transition process by which epithelial cells undergo phenotypic changes, acquire mesenchymal properties and increase their ability to migrate and/or synthesize interstitial matrices is called as EMT. In the lens, this happens commonly after injury as seen in cataract extraction, leading to fibrosis of the lens capsule. Wnt pathway has been shown to be involved in EMT and fibrosis of epithelial cells (He et al. 2009; Konigshoff et al. 2008). Wnt3a, a member of the Wnt family, is found to induce the accumulation of β-catenin and thereby the activation of the canonical Wnt signaling pathway (Nalesso et al. 2011). Transforming growth factor β (TGFβ) has been reported to induce the EMT of lens epithelial cells and promotes Wnt expression during cataract development (Chong et al. 2009).
5.2.9 Advanced Glycation End Products (AGE) in Aging Lens
Proteins when exposed to reducing sugars for a long time leads to accumulation of advanced glycation end products (AGEs) due to nonenzymatic glycation. Proteins like collagens and crystallins, which are long-lived are found to be subjected to this modification. This has been reported as the etiology in diseases like cataracts, diabetic complications, and arteriosclerosis. AGEs may alter cellular functions resulting in fibrosis in lens epithelial cells (Hong et al. 2000).
5.2.10 Antioxidants
Superoxide is converted to hydrogen peroxide by superoxide dismutases, in most tissues of the body, including the lens. Since hydrogen peroxide produces hydroxyl radical OH, it can become highly toxic. Catalase and glutathione peroxidase prevent this toxicity by a system of antioxidant molecules, wherein glutathione plays a vital role (Lou 2003). Ascorbate plays an important part in lens biology, both as an antioxidant and as a UV filter when present in aqueous medium (Varma et al. 1979; Hegde and Varma 2004; Devamanoharan et al. 1991).
5.3 Biochemistry of the Uvea
The uvea is otherwise called as uveal coat/tract or vascular tunic and is comprised of the iris, ciliary body, and choroid, of which the iris and ciliary body lie in the anterior segment, while the choroid spans the entire eye ball between the retina and sclera. The uveal vasculature functions for the nutrition and gas exchange by direct perfusion into the iris and ciliary body and indirectly to the lens, sclera, and retina. Moreover, the uvea helps in reducing the light reflection within the eye, by light absorption, and hence improves the image quality. The most important function of the uvea includes the secretion of aqueous humor by the ciliary process.
The inner surface of the ciliary body which has two layers of epithelium forms the aqueous humor. This epithelium has an inner nonpigmented layer and outer pigmented layer. The ciliary capillaries are highly fenestrated, and the ultrafiltrate of the blood fills the stroma which is formed by the active transport of ions. From the stroma, selective transport of solutes occurs producing osmotic flux producing the AH.
Uveitis is a group of intraocular disorders involving inflammation of the uvea and, in few cases, other related ocular structures that cause about 10 % of blindness (Agrawal et al. 2010), of which about 50 % have bilateral vision impairment (Durrani et al. 2004). Uveitis can be classified as anterior, intermediary, and posterior uveitis; iridocyclitis is the inflammation at the anterior uvea (iris and ciliary body). In case of inflammation of the iris alone, it is referred to as iritis. Inflammation at ciliary body is cyclitis, which is intermediate uveitis. Acute anterior uveitis starts with an intense inflammation around the cornea. The case of posterior uveitis may be bilateral, associated with painless loss of vision. When an inflammation in the vitreous is involved, floaters may be present that are aggregates of inflammatory cells suspended in the vitreous. Loss of vision may be generally due to cataract, opacity in the vitreous, chorioretinal scarring, cystoid macular edema, secondary glaucoma, retinal vascular occlusions, inflammatory optic neuropathy, and retinal detachment (Guly and Forrester 2010; Lowder and Char 1984). Posterior uveitis refers to inflammation in the choroid called as choroiditis, and when the adjacent layer to the uvea is also involved, the condition is termed as retinochoroiditis/uveoretinitis. When the retina is involved, in case of retinal blood vessels, it is called retinal vasculitis, vitritis (vitreous), and when the optic nerve is involved, it is papillitis (Char and Schlaegel 1982).
Various causes have been attributed to the onset of uveitis as drug induced, traumatic, infection induced, and autoimmunity. Uveitis in some cases is found to be generally coupled with many systemic diseases, namely, Behcet’s syndrome, juvenile idiopathic arthritis, sarcoidosis, masquerade syndrome, and infectious diseases like tuberculosis. In about half the cases, it is considered to be idiopathic, and no systemic association is found. In such cases uveitis is presumed to be autoimmune (Rothova et al. 1992). The management of uveitis is determined by the presence or absence of infection or is noninfective and by the likelihood of a threat to sight (Guly and Forrester 2010). The immune response elicited to the infection is considered in part to be responsible for the inflammation and thus the consequent ocular damage and visual loss. Hence topical and systemic steroids are often used in addition to suppress inflammation in patients with infection-related uveitis.
5.3.1 Iris
The human iris is a thin, visible circular structure of eye responsible for controlling the diameter and size of the pupil, thus the amount of light reaching the retina. Iris bounds by the dark pupil and white sclera. It suspends in the aqueous humor behind the cornea but present in front of the lens (Davis-Silberman and Ashery-Padan 2008).
The iris divides the space between the lens and the cornea into the anterior chamber (between the cornea and the iris) and posterior chambers (between the iris and the lens). The periphery of the iris attaches to its root at the iridocorneal angle of the anterior chamber that merges with the tissue of the ciliary body and trabecular meshwork. The human iris formation begins at third month of gestation and completes by the 8 months of gestation, but the pigmentation continues till the first year after birth. The size of the iris varies from person to person with an average size of 12 mm in diameter ranges from 10.2 to 13.0 mm in diameter (Eagle 1988).
The iris consists of four layers:
1.
The anterior layer
2.
Stroma
3.
Sphincter muscle fibers and dilator muscle fibers
4.
The posterior pigment epithelium
The anterior layer is a visible part of the iris which is lightly pigmented. Heavily pigmented melanocytes are present in this layer. The stroma consists of loose connective tissue containing fibroblasts, melanocytes, and collagen fibers. The eye color depends on the melanin pigment content within the stroma and the anterior layer. The involuntary smooth muscle sphincter pupillae or circular muscle lies within the stroma, close to the pupil margin and is about 0.75–1 mm in diameter and 0.1–1.7 mm in thickness.
The dilator pupillae muscles (radial muscles) form a flat sheet beneath the stroma and extends from the iris to the sphincter. These two smooth muscles control the size of the pupil that regulates the amount of light entering the eye.
The posterior epithelium includes two layers of cells that are heavily pigmented. Both anterior and posterior surfaces of the iris provide highly complex and unique iris patterns to individuals. The iris is a well-protected organ, and the iris patterns are stable over many years. Therefore the iris pattern is suitable for individual identification (Eagle 1988).
The important reaction in iris epithelium is formation of melanin pigment. Synthesis of iris melanosome pigment melanin is initiated by converting tyrosine to dihydroxyphenylalanine by the enzyme tyrosinase. Mutation in tyrosinase gene results in ocular albinism.
Recent studies with iris organ culture shows, apart from the differential modulation of tyrosinase transcription, several other factors can contribute to the variability of iris darkening due to certain topical prostaglandin analog therapies. Other enzymes that are involved in the biosynthesis of melanin include 5, 6-dihydroxyindole carboxylic acid oxidase and dopachrome tautomerase. Differential expression of each of these enzymes also alters the formation of melanin. Therefore, variations in each of these serve as potential targets for modulating iridial melanocyte responsiveness to latanoprost (Lindsey et al. 2001).
Iris is the major site to produce prostaglandins that regulates various functions such as smooth muscle contraction, intraocular pressure, and blood-aqueous barrier penetration (Dröge et al. 2003).
Aniridia is a rare, congenital ocular disorder with the characteristics of incomplete formation of the iris caused by the mutations of the paired box gene-6 (PAX6) (Liu et al. 2015).
Fernández-López E et al. reported in their case study first time a sutureless artificial iris implant fixed in the ciliary sulcus after cataract surgery, and they suggest artificial iris will be a promising device for treating photophobia in congenital aniridia (Fernández-López et al. 2015).
5.3.2 Ciliary Body
Human ocular ciliary body (CB) is located between ora serreta and iris which is multifunctional. Ciliary body consists of ciliary muscle and epithelial layer. The epithelial layer is again in two forms, namely, pigmented epithelial layer (PE) and nonpigmented epithelial layer (NPE). The anterior portion of CB continues with iris epithelium; posteriorly NPE continues with neural retina and PE with retinal pigment epithelium. The important function of ciliary epithelium is to produce aqueous humor. Aqueous humor is required to build up the intraocular pressure (IOP) that maintains the eye shape and also nourishes avascular eye tissues, like the lens and cornea. Ciliary muscle helps for proper accommodation of lens (Janssen et al. 2012).
CB synthesizes and expresses multiple neuroendocrine proteins such as neurotensin, natriuretic peptides, and somatostatin, steroid-converting enzymes, transferrin, transthyretin, angiotensin, and growth factors(Coca-Prados and Escribano 2007); these endocrine molecules play role in regulating IOP, the composition of the aqueous humor. The ocular microenvironment of blood-aqueous barrier is anti-inflammatory, minimizes ocular tissue damage, and restores vision clarity (Taylor 2009). The aqueous humor is rich in soluble immunomodulatory factors produced by pigmented epithelium that includes CD86, TGF-beta, and TSP1 (Sugita 2009). NPE and PE also involved in a variety of metabolic process, including lipid and vitamin metabolism showed by gene functional studies. Janssen et al showed the gene expression profiles and functional annotations of the NPE and PE which were highly similar. They found that most important functionalities of the NPE and PE were related to developmental processes, neural nature of the tissue, endocrine and metabolic signaling, and immunological functions. A total of 1576 genes differed statistically significantly between NPE and PE. From these genes, at least 3 were cell specific for the NPE and 143 for the PE. They also observed high expression in the (N)PE of 35 genes that were previously implicated in molecular mechanisms related to glaucoma (Janssen et al. 2012). The CB is also involved in several pathologies. The most important are glaucoma, anterior uveitis/iridocyclitis, (pseudo)exfoliation syndrome, and uveal melanoma (Janssen et al. 2012).
Aqueous humor production depends on the interaction of complex mechanisms within the ciliary body that involves blood flow, transcapillary exchange, and transport processes in the ciliary epithelium.
The enzyme ATPase, present predominantly in nonpigmented epithelium (Cole 1964), is responsible for sodium transport to the posterior chamber and for aqueous formation. It is not a simple dialysate or ultrafiltrate of the blood plasma (Kinsey 1951). An active mechanism demanding high metabolic energy is required for the continuous aqueous production by the ciliary processes.
An ultrafiltration from the capillaries of ciliary processes together with secretory mechanism in the ciliary epithelium produces aqueous humor. The secretory mechanism involves active transport of electrolytes, coupled with fluid transport and carbonic anhydrase action. The produced aqueous humor consists of two chambers of unequal volume, the anterior and posterior and the communication between these two occurs through the pupil. Aqueous humor secreted by ciliary processes flows into the posterior chamber from which it flows into the anterior chamber and drains into extraocular venous systems (McCaa 1982).
5.3.2.1 Trabecular Meshwork
The aqueous outflow determining structure in the anterior chamber includes ciliary muscle and trabecular meshwork (TM). The TM and Schlemm’s canal (SC) are located in the iridocorneal angle. SC is a circumferential channel which accounts for 70–90 % of AH outflow. The endothelial cell lining the SC is the major determinant of the intra ocular pressure (IOP) since it is the primary site of resistance to the AH outflow. It is recently speculated to have features of lymphatic and blood vascular nature (Ramos et al. 2007; Truong et al 2014). The TM is a porous connective tissue (300 × 50–150 μm) with a lamellar arrangement, and the SC is arranged circumferentially (25 mm long) (Hogan et al. 1971). The filtering portion of TM has three regions, namely, uveal meshwork, corneoscleral meshwork, and juxtacanalicular region (JCT). There is also a non-filtering anterior part that protrudes under the cornea (Tamm 2009). The uveal meshwork form irregular intratrabecular fenestrations (Lutjen-Drecoll and Rohen 2001). The trabecular meshwork contains three-dimensional elastic fiber that connects the inner wall of Schlemm’s canal anteriorly, the ciliary body internally, and the choroid and ciliary muscle posteriorly (Overby et al. 2014). The outer layer of cells in TM act primarily as pre-filters and are aggressively phagocytic, removing cellular debris from AH before the fluid moves deeper into the less porous JCT and SC. The endothelial cells play a metabolic role in regulating the AH outflow dynamics. Both adrenergic and sympathetic fibers are present in the TM which increase the outflow facility after adrenoreceptor stimulation (Wax and Molinoff 1987). The contractile property of AH aids in aqueous outflow regulation.
5.3.2.2 Aqueous Humor Formation
Aqueous humor is a transparent watery fluid present in the anterior chamber secreted by ciliary processes of the ciliary body, a musculoepithelial structure, which is located behind the iris. It maintains the intraocular pressure, i.e., the product of the rate of aqueous production and the rate of aqueous drainage. The rate of aqueous humor formation is 2–3 μl/min. The fluid enters into the posterior chamber and flows back through the space between the lens and the iris and enters the nonvascularized tissues of the anterior chamber through the pupil (Rasmussen and Kaufman 2014).
AH forms through passive diffusion, ultrafiltration, and active secretion (Sit 2014). The plasma ultrafiltrate gets accumulated in the stroma which contributes to the AH in the posterior chamber (Civan and Macknight 2004). About 90 % of the aqueous humor formation is through active secretion from the nonpigmented ciliary epithelial cells (Mark 2010). The transport of fluid across osmotic pressure is through the water channel aquaporins (AQPs), namely, AQP1 and AQP4 (Yamaguchi et al. 2006). The ATP required for this transport is supplied by the Na+ /K+ -ATPase, an enzyme located in both the nonpigmented and pigmented ciliary epithelia (To et al. 2001). This can be inhibited by glycosides, vanadate, and acetozolamide and thus gains pharmacological interest (Goel et al. 2010). Another enzyme of pharmacological attention is carbonic anhydrase which is in the nonpigmented and pigmented ciliary epithelia transports bicarbonates. This aids in pH regulation by affecting Na+ ions and helps for active ion transport (Maren 1976).
Apart from electrolytes, carbohydrates, glutathione, urea, proteins, and amino acids are the major constituents in AH. The protein levels are 200 times less in AH compared to plasma, and this is mainly made up of glycoproteins and immunoglobulins like Ig G, Ig M, and Ig A (Allansmith et al. 1973). Free amino acids are present in AH attributing to the active transport (Dickinson et al. 1968). The levels of urea and glucose reflect 80 % of plasma concentration (Reiss et al. 1986). Matrix enzymes like collagenase are also found in AH which helps in the ECM maintenance and contribute to TM resistance pathway (Vadillo-Ortega et al. 1989). Glutathione and ascorbate are the two important antioxidants present in AH, which protect the tissues from light-induced oxidative damage. Ascorbate levels are 50 times more compared to plasma (Leite et al. 2009).
The ciliary, iris epithelium, and the tight junctions of the iris endothelial cells contribute to the blood-aqueous barrier.
5.3.2.3 Aqueous Humor Outflow Pathway
Aqueous humor leaves the anterior chamber through the well-structured tissue, trabecular meshwork, and it reaches the Schlemm’s canal, which drains into the aqueous veins through the conventional pathway (Goel et al. 2010). On the other hand, aqueous humor leaves the eye by diffusion through intercellular spaces among the ciliary muscle fibers through the uveoscleral or non-conventional pathway by diffusion through intercellular spaces among ciliary muscle fibers (Bill 1975). The trabecular meshwork plays an important role in providing most of the flow resistance to aqueous humor outflow. Modification of the trabecular meshwork resistance is responsible for the regulation of intraocular pressure.
Through the trabecular meshwork pathway AH reaches the Schlemm’s canal and drains via the aqueous veins. This flow of AH through TM is dependent on the outflow resistance which is measured as µl/minute/mL of Hg (Stamer and Acott 2012). Through the TM, maximum amount of AH drains. The layers present in the TM are uveal meshwork formed by the iris and ciliary body stroma with large intercellular spaces, followed by corneoscleral meshwork which has lamellar endothelial-like cells which have collagen and elastic fibers. Their intercellular spaces are narrow and offer resistance to aqueous outflow. The next layer is called juxtacanalicular or cribriform meshwork which is rich in extracellular matrix and very narrow intercellular space. The AH has to then cross the endothelial cell lined by the Schlemm’s canal (Epstein and Rohen 1991). This is done by either the paracellular route or transcellular route. There are autonomic and sensory innervations to form neurotransmitters which influence the permeability of TM (Ruskell 1976).
Glaucoma is a condition in which the aqueous humor builds up in the eye leading to increase in the intraocular pressure that consequently damages the optic nerve and nerve fibers from the retina. Normal intraocular pressure ranges from 12 to 22 mmHg. Ocular hypertension is a condition in which the person has high IOP (intraocular pressure) and does not show symptoms of glaucoma. The signs and symptoms of glaucoma include progressive visual field loss, optic nerve changes, and loss of vision which occurs gradually over a long period of time (Tektas and Lutjen-Drecoll 2009). Primary open-angle glaucoma is the most common type of glaucoma (Tektas and Lutjen-Drecoll 2009). People with normal-tension glaucoma have open, normal-appearing angles. In normal-tension glaucoma, optic nerve fibers get damaged even though there is a normal intraocular pressure. It may be considered one of the autoimmune diseases. Primary angle-closure glaucoma is the physical obstruction of trabecular meshwork by the iris (Tektas and Lutjen-Drecoll 2009). Plateau iris glaucoma is a condition where in the anterior chamber, angle closes due to pupillary dilation without iris block. Mixed glaucoma is the combination of primary open-angle glaucoma and primary angle-closure glaucoma. Secondary open-angle glaucoma is characterized by the aqueous outflow resistance between the trabecular meshwork and anterior chamber in pre-trabecular and trabecular form. Glaucomas are treated by applying drops which enhance aqueous draining or reduce aqueous secretion such as beta-blockers to reduce AH secretion (Cheng et al. 2012), alpha-adrenergic agonists (Chew et al. 2014), carbonic anhydrase inhibitors (Scozzafava and Supuran 2014), prostaglandin F2α analogs (Alm 2014), and cholinergic agents or miotic to increase the TM outflow pathway, e.g., pilocarpine and Carbachol (Lee and Higginbotham 2005).
Laser trabeculoplasty is done to treat open-angle glaucoma. A high-energy laser beam is used to open clogged drainage canals and help fluid drain more easily from the eye. Laser surgery for glaucoma initially lowers pressure in the eye. Overtime, however the intraocular pressure may begin to rise (Leahy and White 2015). In trabeculectomy and drainage implants, the fluid is directed to a bleb on the outer layer of the eyeball where it can be absorbed (SooHoo et al. 2014). Laser peripheral iridotomy surgery is used in the treatment of angle-closure glaucoma. In iridotomy, a small hole is created in the iris using a laser so that the fluid can flow through it and leaves the eye. After surgery in the affected eye, the other eye is also recommended for iridotomy because of the high risk that its drainage will also close in the future (Ng et al. 2012).
5.3.2.4 Aqueous Humor Proteomics in Glaucoma
Proteomic analysis of aqueous humor has been done in primary open-angle glaucoma and is suggested as a tool for diagnosis (Izzotti et al. 2010). The proteome can reflect the metabolic changes in the surrounding tissue and therefore can give clues on the pathogenic mechanisms in glaucoma.
TGF-β is known to be elevated in the aqueous humor in all types of glaucoma. This can be secreted from one or more tissues surrounding the anterior segment, including the ciliary epithelium, iris, lens epithelium, corneal endothelium, and trabecular meshwork and it is also possible that the elevated levels of TGF-β in glaucomatous TM tissues could be derived in part from the aqueous humor (Tovar-Vidales et al. 2011).
The differentially expressed aqueous humor proteins in buphthalmic rabbits, a model for “developmental glaucoma,” revealed significant differential expression of several AH proteins in these rabbits based on LC-MS/MS platform (Edward and Bouhenni 2011).
A proteomic analysis of the aqueous humor compositions of “POAG patients” revealed that the protein composition in aqueous humor was significantly different in POAG patients versus non-POAG patients. 2D followed by LC/MS/MS analysis revealed increase in prostaglandins, caspase 14, transthyretin, albumin, cystatin C, albumin, and transferrin (Duan et al. 2010).
Proteomic analysis of the pseudoexfoliation material similarly suggests that extracellular matrix and stress response proteins are associated with “pseudoexfoliation glaucoma” (Lee 2008). Apart from aqueous humor and trabecular meshwork cells, protein profiling at the level of tear has been done that revealed an inflammatory tear protein profile present in “chronically medicated glaucomatous eyes” and are different from that found in primary dry eye. This study describes the drug effect on the treatment profile (Wong et al. 2011).
Gene expression patterns of trabecular meshwork and Schlemm’s canal were shown to be similar to those of circulating leukocytes. The same study reported on the extensive alterations in characteristic protein expression patterns of circulating leukocytes for “normal-tension and primary open-angle glaucoma” (Golubnitschaja et al. 2007; Zhao et al. 2004).
5.3.2.5 Aqueous Humor Metabolites in Glaucoma
Reactive oxygen species (ROS) play a fundamental role in the pathophysiology of many diseases, including glaucoma. The iron-catalyzed formation of ROS is a major player in these processes. Erdumus et al have reported decreased serum antioxidants and increased serum superoxide dismutase (SOD) levels in patients with POAG indicating that antioxidant system dysfunction may be involved in the etiopathogenesis (Erdurmus et al. 2011). Studies have found that hepcidin, a prohormone produced in the liver, is expressed in other eye tissues, such as in Muller cells, photoreceptor cells, and retinal pigmented epithelium (RPE). It is increased in aqueous humor of POAG patients, with an expression pattern similar to that of ferroportin (Sorkhabi et al. 2010). It is also widely accepted that mitochondria might play a pivotal role in the pathophysiology of glaucoma (Kong et al. 2009). Low citrate has been reported in the plasma of glaucoma patients (Fraenkl et al. 2011). Citrate is an important molecule in the energy production cycle in mitochondria, and reduced mitochondrial activity might also lead to a decreased citrate production resulting in lower blood citrate levels. Oxidative DNA damage may induce human trabecular meshwork degeneration, leading to increase IOP. Increased levels of 8-hydroxy deoxyguanosine, (8-OHdG), an indicator of DNA damage, was seen in human trabecular meshwork specimens collected from patients with primary open-angle glaucoma (POAG) (Sacca et al. 2005). Patients with PACG also had high 8-OHdG concentrations (Chang et al. 2011). Apart from oxidative stress and DNA damage, aqueous amino acids levels are also reportedly altered. Individual amino acids, namely, threonine, serine, asparagine, glutamine, methionine, tyrosine, phenylalanine, histidine, tryptophan, and arginine, were reported to be higher in aqueous of glaucomas than in the cataract patients (Hannappel et al. 1985). Plasma levels of homocysteine were also increased in pseudoexfoliative glaucoma (PXG) patients and POAG patients (Turgut et al. 2010). Higher levels of hydroxyproline reported in aqueous indicative of increased collagen turnover is reported in pseudoexfoliation syndrome (PXF) in syndrome (Yagci et al. 2007).
5.4 Biochemistry of Sclera
The sclera is the white, fibrous, opaque protective external tunic of the eye rich in elastin and collagen fibers. It extends from the anterior to posterior chambers, i.e., limbus (corneoscleral junction) to the cribriform plate (optic nerve head). The type I collagen irregularity contributes to the opaqueness of the sclera (Keeley et al. 1984). The layers of sclera are episclera, stroma, lamina fusca, and endothelium. The sclera is continuous with the dura mater and cornea. It contributes to the maximal connective tissue layer of the eye, providing resistance against the internal and external stresses and acts as a support harboring extraocular muscular insertions. A healthy sclera appears normal and white, while one with inflammation has the scleral blood vessels engorged. While the episclera is vasculairsed, the scleral stroma is avascular receiving the nutrients from the episcleral and he underlying choroidal vasculatures. The sclera is richly supplied with nerves. The posterior ciliary nerves enters the sclera near the optic nerve.
The metabolic requirement of the sclera is low and has low turnover of the collagen. Scleral matrix is made up of scaffold of protein fibrils, collagen and elastin, and interfibrillar proteoglycans and glycoproteins, which surround a diffuse population of cells. The human sclera contains predominantly collagen which is about 50–75 % depending upon the different techniques done for estimating (Keeley et al. 1984).
The Tenon’s capsule fibroblasts synthesize the collagen (Gross 1999). These include the different types of collagen such as I, II ,V, and VI with type I followed by type III as the predominant ones, and are restricted to the outermost sclera (Heathcote 1994). The collagen fibrils of the sclera range from 25 to 300 nm as observed by electron microscopy (Komai and Ushiki 1991). There are thinner fibrils in the lamina cribrosa, trabecular meshwork, and corneoscleral angle (Albon et al. 1995). This is attributed to adaptation and the required elasticity (Parry and Craig 1984). Type XII is associated with type I fibrils in human sclera as a different isoform (Anderson et al. 2000). The elastin component of the sclera aids as additional supporting system for the collagen network. Elastin contains amino acids like alanine, valine, isoleucine, and leucine. It contains little hydroxyproline and hydroxylysine unlike collagen. It contains cross-links, namely, desmosine and isodesmosine. The elastin fibers are abundant in the inner stromal region near the extraocular muscles. There are four times more elastin in the lamina cribrosa and peripapillary sclera compared to the equatorial region (Quigley et al. 1991). The sclera matrix consists of proteoglycans. The main proteoglycans mostly belong to small leucine-rich repeat family members, namely, Decorin and Biglycan (Friedman et al. 2000). Decorin has been found to play an important role in development and wound healing, and reduced synthesis is found and seen in the development of myopia (Rada et al. 2000). Lumican found in the sclera by immunohistochemical studies is involved in regulating collagen fibrillogenesis and has been linked with high myopia in humans. Lumican deficiency causes scleral anomalies (Austin et al. 2002). Recent studies have revealed the presence of perlecan, agrin, prolargin, versican, aggregan, mimecan, and fibromodulin in scleral layers as revealed by immunofluoresence staining (Keenan, et al. 2012). These studies reveal that electron dense filaments of the decorin distribute along the collagen fibrils with a periodic pattern (Kimura et al. 1995). The C-terminal region of collagen type I binds to decorin and is also associated with type VI collagen (Keene et al. 1987).
Sclera exhibits low cellularity compared to most vascularized tissues, and the only cell is the scleral fibrocyte. Sclerocytes can undergo rapid transformation into active fibroblasts following insult like trauma, surgery, postoperative scarring and topical application of drugs, and neoplasia to the sclera. Histiocytes, blast cells, granulocytes, lymphocytes, and plasma cells can also be seen in the sclera occasionally. In inflammatory stimulus, these cells enter from blood vessels of the choroid and episclera and arrive to the site of insult. Scleral cells respond to growth factors like platelet-derived growth factor (PDGF), transforming growth factor, fibroblast growth factor, thymocyte-derived growth factors, IL-1 and interferon gamma.
Scleritis is a chronic ocular inflammatory condition of the sclera. Scleritis is marked with redness in the sclera that is diffuse or localized in a pie-shaped area. The etiology of this condition varies from idiopathic, confined to the eye to systemic autoimmune diseases and in rare cases due to infection or drug reaction as well as due to a tumor or surgical complications. Infectious scleritis can be primary or secondary to keratitis or endophthalmitis. Surgery, trauma, and the use of corticosteroids are considered as major predisposing factors (Ramenaden and Raiji 2013; Smith et al. 2007). With non-necrotizing scleritis, there is no much threat to vision unless there is presence of uveitis or keratitis. In case of necrotizing scleritis, much visible necrosis of sclera can be viewed along with the exposure of the underlying uvea, which is more often coupled with rheumatoid arthritis or systemic vasculitis (Smith et al. 2007; Sainz de la Maza and Foster 1994). Watson and Hayray (Watson and Hayreh 1976) defined five distinct classifications of scleritis, namely:
(a)
Anterior scleritis which involves inflammation in the anterior sclera that can be diffused or nodular or can be non-inflammatory necrotizing scleritis
(b)
Scleromalacia perforans: Inflammatory necrosis of anterior sclera
(c)
Posterior scleritis: Inflammation of posterior sclera
(d)
Sclerokeratitis: Inflammation of sclera that occurs in association with cornea
(e)
Episcleritis: Inflammation of sclera and conjunctiva
5.4.1 Molecular Derangement in Uveitis
Uveitis phenotypes can differ significantly, and most types of uveitis are believed to be polygenic with complex inheritance patterns. In uveitis there is a strong association of HLA-B27 with acute anterior uveitis and HLA-A29 with birdshot chorioretinopathy (Martin et al. 2003). The genomic analysis of serum, aqueous, and vitreous of Fuchs uveitis-affected patients showed the presence of rubella virus-specific antibody and its gene, speculating the probable involvement of the virus in chronic inflammation of this disease (Cimino et al. 2013). Studies suggest that uveitis shares some of its pathogenic mechanisms associated with other autoimmune diseases and conclude the “common gene, common pathway” hypothesis for autoimmune disorders (Mattapallil et al. 2008). The study involving rat model of experimental autoimmune uveitis (EAU) recognized three quantitative trait loci (QTL) linked with EAU on rat chromosomes 4, 12, and 10 (Eau1, Eau2, and EAU2) (Pennesi and Caspi 2002). Using microsatellite markers of resistant and susceptible MHC class II-matched rat strains, new possible QTLs on rat chromosomes 2, 3, 7, 10, and 19 (Eau4-Eau9) were identified that direct susceptibility to uveitis. Also a protective allele was identified in the uveitis-susceptible rat strain in the Eau5 locus at D7Wox18, and epistatic interactions between QTLs influenced the severity. These identified regions were found to colocalize with the genetic determinants of other autoimmune disease models and to the syntenic regions recognized on human chromosomes 4q21-31, 5q31-33, 16q22-24, 17p11-q12, 20q11-13, and 22q12-13, from patients suffering from autoimmune disorders.
Autoimmune uveitis encompassing inflammatory triggers occurs with relapse. The mechanism of the reaction and the molecular agents eliciting relapse has remained unexplored. To understand this, studies have used the only spontaneously occurring uveitis model equine recurrent uveitis (ERU), for proteomics and molecular analysis, since it very closely resembles the human autoimmune uveitis. In autoimmune uveitis, autoreactive T cells cross the blood-retinal barrier and damage the inner eye tissues. Studies have identified CRALBP (cellular retinaldehyde-binding protein) as a novel antigen (Deeg et al. 2006). Anti-CRALBP antibody was significantly elevated in the sera of affected individuals (Deeg et al. 2007c). Malate dehydrogenase, IRBP (iron-responsive element binding protein), the highly antigenic S-antigen (aka S-arrestin) and recoverin are the other identified proteins (Deeg et al. 2007b, 2008). Increase in VEGF and lowered PEDF (pigment epithelium-derived factor) with blood-retinal barrier breakdown aid autoreactive T cell infiltration (Deeg et al. 2007a). Increased incidences of IgG4 hc, IgM, alpha-2HS-glycoprotein, serotransferrin, and complement factor B, with decreased levels of IgG5 hc, apolipoprotein A-IV, apolipoprotein H, and high-molecular-weight kininogen, are reported (Zipplies et al. 2010). Based on immunohistochemistry analysis, increase in the complement activation products B/Ba, B/Bb, Bb neoantigen, iC3b, and C3d with no difference in C5b-9 levels is reported. Macrophage involvement in the inflammatory process of autoimmune uveitis is reported (Zipplies et al. 2010). Additionally differential expression of several cellular proteins such as MMPs and TIMP-2 (matrix metalloproteinase and their inhibitors) and potassium ion channels and decreased osteopontin and fibronectin have been reported (Deeg et al. 2011; Eberhardt et al. 2012; Hofmaier et al. 2011; Zayas-Santiago et al. 2014).
5.5 Biochemistry of the Vitreous, Retina and Retinal Pigment Epithelium
5.5.1 The Vitreous
The vitreous humor occupies a large portion of the eye posteriorly: behind the lens and in front of the retina. It is a clear jelly-like substance that fills the eye and gives a shape. The vitreous is divided into three compartments, namely, vitreous base, central vitreous, and cortical vitreous. Concentration and arrangement of collagens differ in all the three layers. The concentration of collagen is about 300 μg/ml and it is highly insoluble. It contains predominantly type II collagen with lower amounts of type IX, type V/XI collagen (de Smet et al. 2013). Collagen is arranged from anterior to posterior direction with denser arrangement of collagen fibers in the vitreous base and cortical vitreous, while the central part has very thin collagen fibrils. The vitreous base covers the dorsal side of the lens, and ends at the region of ora serrata, where the neurosensory retina begins. The cortical vitreous begins at the retina and maintains the strong attachment through inner limiting lamina (ILL). Collagen fibers run parallel to the ILL and acts like strong glue over the retina, to spread over the RPE. The less dense central vitreous makes the bulk of vitreous zone with the collagen fibers running from anterior to posterior direction from anterior to posterior part (Bishop 2000; Le Goff and Bishop 2008). Hence, this arrangement of collagen fibers making a contact over the inner surface acts like a substratum for lens ciliary body and retina. It also acts as a shock absorber.
The vitreous is composed of 98–99 % water with collagen gel fibril and in between with a network of very long molecules of hyaluron (HA) which render the viscoelastic property to the vitreous (Swann 1987). The viscoelastic property of the vitreous is required for protecting the retina from shock. The hyaluronic acid and collagen interaction gives transparency and hydration and the spacing arrangements decrease light scattering in the vitreous (Bishop 2000).
The vitreous contains heterotypic collagen fibril formed by the co-assembly of type II, IX, V, and XI collagen. Among the total collagen content of the vitreous, type II constitutes (60–75 %), type IX (25 %), type V, VI, and XI collagen constituting minor quantities. These collagenase core is bound with HA attracts the counter ions and water and swells the vitreous. It also space the collagen fibers apart from clumping and makes the vitreous a transparent medium for the passage of light. Chondroitin sulfate is also found in the vitreous, decorating type XI collagen. There are also few noncollagenous proteins present in the vitreous, namely, albumin, transferrin, lactoferrin which can be seen in gel electrophoresis (Nguyen and Fife 1986).
The glycosaminoglycan present in the vitreous is hyaluronic acid with a molecular weight of 3000 kDa. The HA-collagen interaction comes from the electron microscopic observation of vitreous. These interactions are found to occur through chondroitin sulfate chains and collagen fibrils (Theocharis et al. 2008). Concentration of HA in the human vitreous is between 65 and 400 μg/ml (Balzas 1984).
There are a few cells in the vitreous called as hyalocytes which are more towards the peripheral part of the vitreous. They are star-shaped stellate cells with oval nuclei measuring 10–15 μm (Sommer et al. 2009) and are considered as specialized form of macrophages. They are known to synthesize extracellular matrix and regulate vitreous immunology in inflammation. The hyalocytes play a major role as antigen-presenting cells (Sakamoto and Ishibashi 2011). In vitro the hyalocytes can be cultured, and they response to inflammatory molecules by secreting urokinase-type plasminogen activator and vascular endothelial growth factor (Hata et al. 2008). Hyalocytes are present in epiretinal membrane and contribute to the inflammatory mechanism (Joshi et al. 2013).
Formation of Vitreous Humor
Separation of neural and surface ectoderm at 3rd to 4th week of gestation forms the vitreous cavity and it is filled with collagenous-like material. Mesodermal cells enter into this vitreous cavity, forms hyaloid vessels, and it is branched throughout the vitreous cavity. Fibroblast and phagocyte cells from the outer most layer of this vessel latter differentiate into hyalocytes. A cellular-material-like collagen formed after 6th week of gestation. Hyaloid vessels regress as the fetus develops and are not seen in adults (Jack 1972; Linsenmayer et al. 1982).
< div class='tao-gold-member'>
Only gold members can continue reading. Log In or Register a > to continue
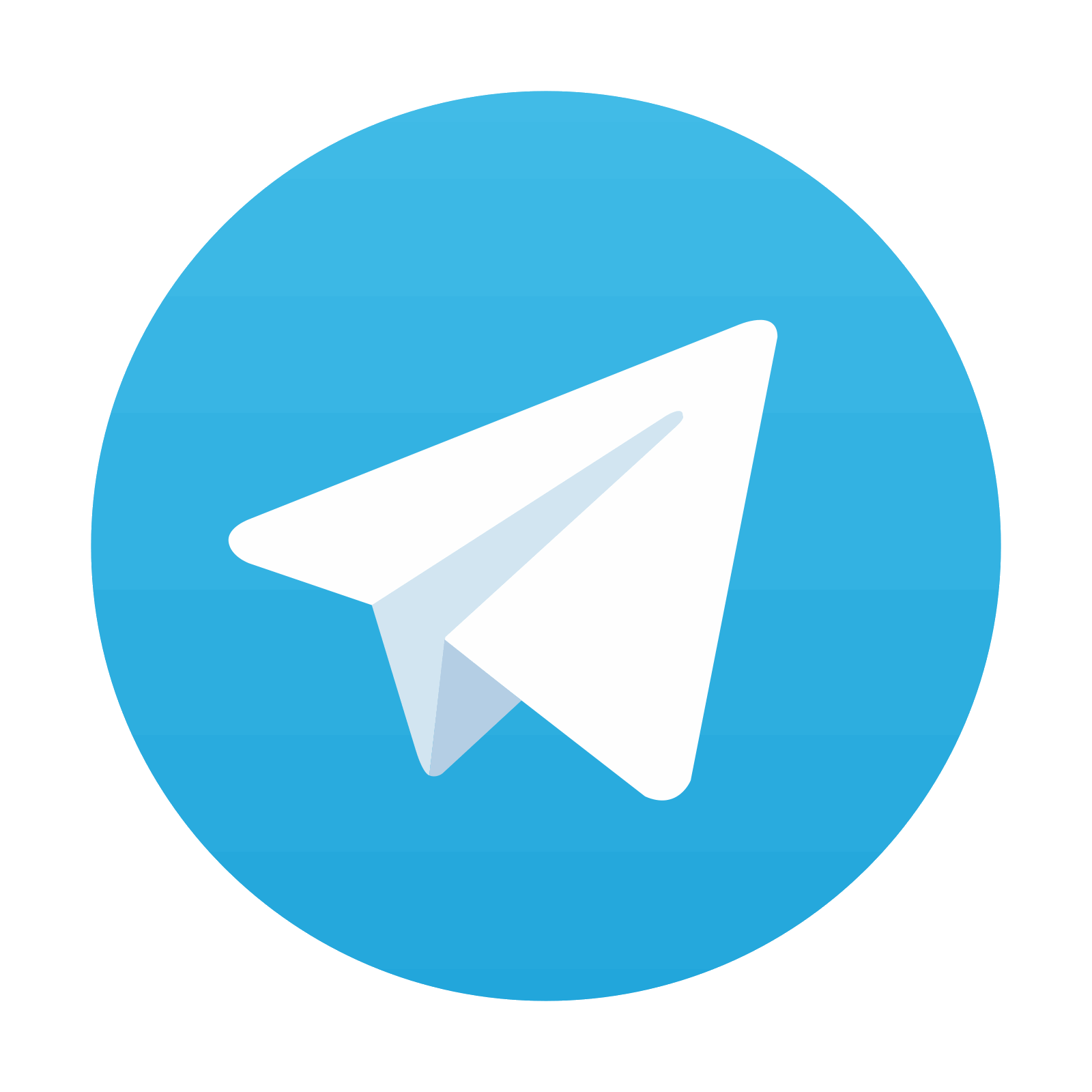
Stay updated, free articles. Join our Telegram channel
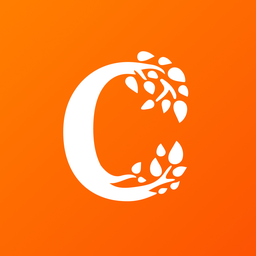
Full access? Get Clinical Tree
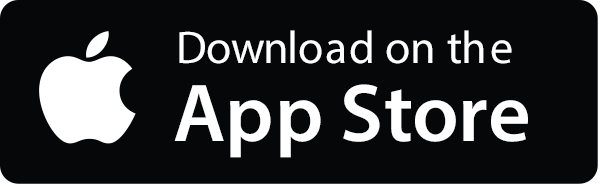
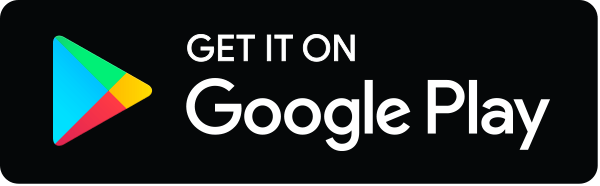