Fig. 19.1
Anatomy of the eye and the biological barriers of the eye (tight barriers are indicated in red, others in green; route of elimination). The main pathway for drugs to enter the anterior chamber is via the cornea (Lee and Robinson 1979). Some large and hydrophilic drugs prefer the conjunctival and scleral route and then diffuse into the ciliary body (Maurice and Mishima 1984). After systemic administration, small compounds can diffuse from the iris blood vessels into the anterior chamber (Young and Heath 2000). From the anterior chamber, the drugs are removed either by aqueous humor outflow (Barar et al. 2008) or by venous blood flow after diffusing across the iris surface (Klyce and Crosson 1985). After systemic administration, drugs must pass across the retinal pigment epithelium or the retinal capillary endothelium to reach the retina and vitreous humor (Sasaki et al. 1999). Alternatively, drugs can be administered by intravitreal injection (Huang et al. 1983). Drugs are eliminated from the vitreous via the blood–retinal barrier (Huang et al. 1989) or via diffusion into the anterior chamber (Dartt 2002) (Reproduced with permission from Hornof et al. 2005)
19.1.1.1 Corneal Barrier
The topical ocular route of administration is preferred for many classes of drugs due to ease of access and patient compliance when treating diseases of the anterior segment. The topically applied drugs are rapidly eliminated from the precorneal area. In the precorneal area, solution drainage, tear dilution, tear turnover, tear fluid-enabled lacrimal drainage, and the conjunctival absorption allow only a small fraction of the drug to be absorbed into the eye (Lee and Robinson 1979; Maurice and Mishima 1984). The cornea (transparent tissue) is a front part of the eye, and it can be called as a window of the eye is considered as a major pathway for topical drugs. It provides an important mechanical and chemical barrier, which limits the access of exogenous substances into the eye and protects the intraocular tissues. The cornea is made up of five layers: corneal epithelium, basement membrane, Bowman’s layer, stroma, Descemet’s membrane, and endothelium (Fig. 19.2) (Young and Heath 2000; Barar et al. 2008). The corneal epithelium constitutes flattened superficial cells, wing cells, and columnar basal cells. The superficial cells are in close contact with one another via desmosomes, and these cells are surrounded by annular tight junctions–zonulae occludentes (Klyce and Crosson 1985; Sasaki et al. 1999). The tight junctions of the corneal epithelium are the rate-limiting barrier for hydrophilic drug permeation, whereas lipophilic drugs have comparatively higher permeability. The stroma and endothelium offer little resistance as compare to epithelium for transcorneal permeation (Huang et al. 1983, 1989). The stroma constitutes 85–90 % of the total corneal mass and is mainly composed of hydrated collagen. Stroma’s hydrophilic nature exhibit a diffusional barrier to highly lipophilic drugs (Huang et al. 1983). The innermost layer of cornea–corneal endothelial monolayer conserves an effective barrier between the stroma and aqueous humor.
19.1.1.2 Conjunctival Barrier
Topical drugs reach various ocular structures through corneal and/or the non-corneal conjunctival (non-corneal) pathways (Fig. 19.1). The conjunctiva’s greater surface area (17 times larger than human cornea) and relatively weaker epithelium provides opportunity for better topical absorption of drugs. It covers anterior one-third of the eye ball. The conjunctiva consists of an outer epithelium and beneath that lies the stroma. Microvilli cover the epithelium, and they are composed of 5–15 layers of stratified epithelium. Tight junctions connect the adjacent epithelial cells on the apical side due to which it exerts the intercellular drug permeability barrier. The stromal layer which attaches itself to the sclera has nerves, lymphatics, and blood vessels (Dartt 2002).
The palpebral conjunctiva lines the inner surface of the eyelids and bulbar conjunctiva which covers the anterior surface of the sclera. The bulbar conjunctiva represents the first barrier for the non-corneal permeation of topically applied drugs. Conjunctival route is favored for hydrophilic and large polar molecules bypassing anterior segment and having direct access to posterior segment tissues (Hosoya et al. 2005). The drugs can cross the conjunctiva through paracellular or transcellular routes. Also the drugs which are substrates of the influx transporters are reported to have been transported using the transporters (Zhang et al. 2006; Garrett et al. 2008). However, the transporter-mediated transport in conjunctiva has not been explored much. The pore diameter and pore density was reported to be 2 and 16 times more in conjunctival epithelium than corneal epithelium of rabbits. This also could be the reason for higher drug permeability than cornea (Hamalainen et al. 1997). Another important thing to consider is the conjunctival blood vessels; a significant amount of topical and subconjunctivally injected drugs will be lost to the conjunctival blood circulation (Chang and Lee 1987; Urtti and Salminen 1993). Further details about cellular and metabolic functions various parts of the eye are explained in detail in the Chapter of Ocular Biochemistry (Chap. 5).
19.1.1.3 Blood–Aqueous Barrier (BAB)
The BAB is located at the anterior portion of the eye, and the access of drugs to the aqueous humor of the anterior and posterior chambers is restricted by the BAB (Figs. 19.1 and 19.2). The BAB executes its barrier functions by the presence of nonpigmented cell layer of the ciliary epithelium, posterior iridial epithelium, and endothelium of the iridial blood vessels. These structures are reported to have leaky tight junctions as compared to BRB (Cunha-Vaz 1979). The BAB regulates the solute exchange between the blood and the intraocular fluids – hence limits the entry of plasma albumin/drugs into aqueous humor (discussed in detail in Chap. No.3). However, some pathological conditions like inflammation could disrupt this barrier and allow the improved entry of drugs (Urtti 2006).
19.1.1.4 Blood–Retinal Barrier (BRB)
BRB at the posterior segment of the eye consists of inner BRB (iBRB) and outer BRB (oBRB) (Figs. 19.1 and 19.2). The iBRB and oBRB constitute endothelial membrane of the retinal blood vessels and retinal pigment epithelium (RPE). The cellular tight junctions “zonula occludens” seal off the spaces between the RPE cells (Cunha-Vaz 1976). Both the inner and outer BRB offer significant restriction to the permeability of drugs (discussed in detail in Chap. 3). The diffusion (permeability) of drugs across BRB is determined by its lipid solubility. BRB due to its specific permeability characteristics provide its potential role in the pathophysiology and posterior therapeutics of diabetic retinopathy (DR), age-related macular degeneration (ARMD), and diabetic macular edema (DME) (Cunha-Vaz 1976; Mannermaa et al. 2006). It separates blood from neural retina. The oBRB being a tight ion-transporting barrier restricts paracellular transport of polar solutes from the choroidal side. The iBRB offers significant resistance to systemic penetration of drugs. A drug should possess optimum membrane partition characteristics or should be a substrate for one of the membrane uptake transporters present on the iBRB or oBRB to cross BRB (Cunha-Vaz 1976; Nirmal et al. 2012, 2013a, b). Both BAB and BRB contain epithelium and endothelium with tight junctions that restrict the entry of drugs through paracellular route (Cunha-Vaz 1979; Rizzolo et al. 2011).
19.1.2 Various Routes of Ocular Drug Delivery
To achieve a therapeutic success for various ocular diseases, various possible routes of drug delivery are being used (Fig. 19.1). However, the selection of administration route mainly depends on the targeted site of action. Conventionally, topical, intravitreal, systemic (oral and intravenous), and recently periocular routes are used. To treat the anterior segment of the eye, mostly topical route is used, whereas for posterior segment, intravitreal and periocular routes are used. Systemic route has been used traditionally for treating both anterior and posterior segment (Urtti 2006). Ocular pharmacokinetics of drugs administered through various routes are explained in Chap. 3.
19.1.2.1 Topical Drug Delivery
The most patient compliant and the conventional route is topical delivery into the cul-de-sac using eye drop. In humans, the contact time of the eye drop in the precorneal area is very less (less than 5 min), and it also activates lacrimation and tear turnover which dilutes the drug concentration (Hughes et al. 2005). Hence, less than 1 % reaches the aqueous humor (Lee and Robinson 1986; Mikkelson et al. 1973). During this short time, the drugs which are small ionic and hydrophilic enters the anterior chamber through the pores (60 A°) of the corneal epithelium (Lee 1990). In case of lipophilic drugs, the drug remains in the epithelium due to its lipid solubility and release to the stroma and then to the anterior chamber (Sieg and Robinson 1976). The drug reaches its maximum concentration at 20–30 min in aqueous humor. From there, it gets distributed to the iris and the ciliary body (Maurice and Mishima 1984; Urtti et al. 1990). The elimination from aqueous humor is through the aqueous turnover, Schlemm’s canal, and the anterior uveal venous blood flow (Maurice and Mishima 1984). These mechanisms render the overall half-life of topical eye drops in the anterior chamber in around 1 h (Urtti 2006). Hence, a topical delivery requires frequent administration with high concentration. There is a real need for the topical delivery system which could release the drug for extended period without frequent administration.
19.1.2.2 Systemic Drug Delivery (Oral and Intravenous)
For oral drugs, the ocular bioavailability is determined by the plasma concentration it reaches after the intestinal absorption. The intraocular penetration determined by the drugs’ physiochemical and transporters susceptibility at ocular barriers (Nirmal et al. 2012, 2013a, b; Velpandian 2010). Both BAB and BRB restrict the entry of systemic drugs. The systemic drugs enter the anterior segment by penetrating the leaky vessels of the ciliary body and diffuse through the iris to aqueous humor (Cunha-Vaz 1979). In the posterior segment, the drugs which could reach choroid will be cleared by choriocapillaris (densely packed network) – luckily, it has numerous fenestrations in the endothelium which offers less resistance. However, the further movement into vitreous is restricted by RPE except for the compounds with extreme lipophilic nature. Additionally, the functional transporter systems present in the RPE and retinal vessels efflux the drugs out from vitreous to plasma (vitreous elimination) which renders the short half-life of the drugs in vitreous. As a result, most of the compounds with good pharmacological activity reaches poorly inside the eye, hence requiring high systemic concentration which provides unwanted side effects and poor therapeutic success. Hence, systemic drug delivery systems with specific targeting are highly needed, without which only a fraction can reach after oral or intravenous administration (Urtti 2006; Nirmal et al. 2013b).
19.1.2.3 Intravitreal Drug Delivery
To overcome the BRB and achieve the required therapeutic concentration inside the vitreous chamber, direct vitreal administration is a more straightforward approach. In many posterior segment diseases like DR, ARMD, and DME, vitreous administration is still the mainstay of treatment (Hughes et al. 2005). However, the intravitreal administration has its own disadvantages like frequent administration, hemorrhage, retinal detachment, and endophthalmitis (Jay and Shockley 1988; Daily et al. 1973). The drug gets eliminated from vitreous through both the anterior and posterior segment. The anterior elimination occurs by diffusion from vitreous to posterior chamber and elimination via aqueous turnover and uveal blood flow (Maurice and Mishima 1984). Recently, it has been revealed that the cationic drugs predominantly undergo anterior elimination using organic cation transporters (Nirmal et al. 2012). The posterior segment elimination occurs due to permeation across BRB through passive permeability or by active efflux transporters (Maurice and Mishima 1984; Senthilkumari et al. 2009; Hosoya et al. 2009). Hence, large or hydrophilic molecules tend to have longer vitreal half-life. In the vitreous, the mobility of large molecules is restricted, especially the positively charged; however, the small drugs could diffuse rapidly (Pitkanen et al. 2003). The influence of compromised BRB in various disease conditions in altering the vitreal drug pharmacokinetics needs further studies and serious consideration. The drug delivery system like injectable implants which could deliver the drugs for prolonged period of time holds the promise for intravitreal therapeutics.
19.1.2.4 Periocular Drug Delivery Systems
Periocular denotes the periphery of the eye or surrounding region of the eye. Periocular drug delivery could be achieved via subconjunctival, sub-Tenon, peribulbar, posterior juxtrascleral, and retrobulbar spaces (Ranta and Urtti 2006). The sclera due to its large surface area provides potential advantage for periocular drug delivery. Periocular drugs could reach posterior segment through the anterior chamber route, systemic circulation route, and direct penetration route (Eljarrat-Binstock et al. 2010). Periocular drug delivery systems need to overcome the episclera, sclera, choroid, Bruch’s membrane, and RPE before reaching the vitreous humor or the retina (Kim et al. 2007; Ghate et al. 2007). This could be the longest and most problematic route to reach vitreous and retina due to the presence of static barrier and dynamic clearance mechanisms (Ghate and Edelhauser 2006). Potential capabilities of new novel drug delivery systems could provide a new importance to this route for treating various posterior segment diseases. However, detail studies are still needed to understand the pharmacokinetics of the periocular drug delivery systems from various periocular spaces before questioning its future.
19.1.3 Importance of Understanding Blood–Ocular Barriers to Overcome It Using Drug Delivery Approaches
The clinical success of ophthalmic therapeutics was reported to be approximately 14 % (Kola and Landis 2004). Ophthalmic drugs are one among the various areas have very limited clinical success as compare to other therapeutic areas. This scenario could be due to: (i) no/very few ocular-specific drugs are being developed and (ii) lack of sufficient understanding of blood–ocular barriers to overcome it using appropriate drug delivery systems. The ocular barriers exert its property through (i) regulated diffusion through the paracellular spaces, (ii) transcellular facilitated diffusion, (iii) transcellular active transport, (iv) transcytosis, and (v) metabolic processing (Rizzolo et al. 2011). The contribution of each of these pathways in the drug transport across ocular barriers is not clear. However, the transport of majority of the drug molecules/solutes through various ocular epitheliums occurs by either through the cells (transcellular) or between the cell (paracellular) routes. Generally, hydrophilic drugs have less penetration across various ocular epithelium; hence, they require some active uptake process/transporter for their penetration or transport through paracellular route (Mannermaa et al. 2006; Hughes et al. 2005; Ueda et al. 2000). The size and charge of the intercellular spaces determines the transport of drugs through the paracellular route. The paracellular permeability is limited by four types of cell–cell junctions which include tight junctions (TJ), adherens junctions, gap junctions, and desmosomes in vertebrates. In 1963, the tight junction or zonula occludens was discovered by Farquhar and Palade (1963). The TJs are formed just beneath the apical surface in epithelial cells (to seal neighboring cells) to determine transport of solutes like water, ions, drugs, and other molecules (Farquhar and Palade 1963). The drug/molecule with radii up to 15 A° can cross through the tight junctions. The cells attain the mechanical stability by zonula adherens and desmosomes. Electrical and metabolic coupling are ensured by gap junctions (Simon and Goodenough 1998).
The lipophilic drugs cross the epithelium due to their lipid solubility in the cell membrane lipids. Drugs with specialized transport process utilize the transcellular route (Ho and Kim 2005). The drug interaction with the cell is influenced by both drug and cell characteristics. The transcellular transport mechanisms include passive diffusion, facilitated diffusion, and receptor-mediated endocytosis. These mechanisms are implemented by the cells using the membrane proteins (transporters or receptors). The membrane transporters (so-called drug transporters) are the militants located across the eye. Many of the ocular drugs are substrates of these transporter proteins. It is also very clear that these transporters share a substantial percentage in the ocular pharmacokinetics and disposition of drugs (Mannermaa et al. 2006). Hence, there is a potential opportunity for the drug delivery systems to target the drug transporters to optimize the ocular bioavailability of therapeutics.
Drug transporters could enhance or prevent the entry of drugs across blood–ocular barriers. Based on these characteristics, transporters are divided into uptake (influx) or export (efflux) of the drugs which are their substrates – hence, it could be an opportunity or barrier for drug delivery. Apart from the physicochemical characteristics of the drugs (molecular size, lipophilicity, and the pKa), these transporters determine the transport of the drugs across various ocular barriers. The various ocular barriers are known to express several of these transporters at both apical and basolateral surfaces (Nirmal et al. 2012, 2013a, b; Zhang et al. 2008; Vadlapatla et al. 2014). However, the percentage contribution of various factors is not very clear yet and needs further studies. The transporters could also provide mechanistic details of treatment failure, individual response to the treatment, and adverse drug reaction (Tamai and Tsuji 2000).
The two major superfamilies ATP-binding cassette (ABC) and solute carrier (SLC) transporters consist of 400 membrane transporters (International Transporter Consortium et al. 2010; Schlessinger et al. 2013). Efflux and influx transporters belong to the ABC and SLC superfamily. The various transporters’ localization in ocular tissues is reported in (Zhang et al. 2008; Vadlapatla et al. 2014). Efflux transporters reduce intracellular concentration of drugs by expelling it out of the cell, and it operates as a unidirectional transporters. Till now 48 ABC transporters belonging to seven different classes (ABCAABCG) have been identified (Hediger et al. 2004; Vadlapatla et al. 2014). Among them P-glycoprotein (P-gp/MDR1), multidrug-resistant proteins (MRPs), and breast cancer-resistant protein (BCRP) are considered important. Influx transporters help to transport nutrients, peptides, ions, peptides, metabolites, other endogenous amines, and exogenous molecules. In human genome, 386 SLC transporters belonging to 43 different families (SLC1-SLC43) have been identified. Among them organic cation transporters (OCT/OCTN), organic anion transporters (OAT/OATP), peptide transporter (PEPT), monocarboxylate transporters (MCTs), amino acid transporters, and sodium-dependent multivitamin transporter (SMVT) (Vadlapatla et al. 2014).
Transporter-targeted drug delivery could become a clinically significant drug delivery approach (Duvvuri et al. 2004). P-gp and MRPs were the most explored transporters in the eye. Mitra group have demonstrated the importance of inhibiting P-gp and MRPs to improve the ocular bioavailability of various clinically important drugs (Vadlapatla et al. 2014). Several transporters or receptors for nutrients and endogenous compounds are expressed in the cornea, conjunctiva, and RPE representing opportunities for ocular delivery of drugs. For example, corneal permeability of antiviral drugs such as acyclovir and ganciclovir is improved when converted into their L-valyl esters which could be due to targeting dipeptide transporters in the cornea (Anand and Mitra 2002). Evidence suggests that the presence of peptide transporters (PEPT1 and PEPT2) in the cornea is involved in the absorptive mechanism for their drug substrates after topical dosing to the cornea (Zhang et al. 2008). Few of the studies have also reported the utilization of BRB for enhanced nonviral gene transfer, utilizing transporters like folate and vitamin C transporters/receptors located in the RPE (Lee and Huang 1996; Guo and Lee 1999). An oligopeptide transport system is involved in the transport of a model dipeptide after systemic administration across ocular barriers (Atluri et al. 2004). Carrier-mediated transport process for brimonidine in the RPE has been demonstrated in bovine RPE-choroid explants and polarized human adult retinal pigment epithelial cells (ARPE-19), suggesting its role in modulating the movement of brimonidine into and out of the eye (Zhang et al. 2006). Organic cation drug absorption could be greatly enhanced by targeting OCT in the cornea and BRB (Nirmal et al. 2012, 2013a, b). Transporter-mediated drug penetration present at iBRB would confer great advantage over passive diffusion due to the tight barrier of the retinal endothelium. Therefore, it is important to design and select optimal drug candidates by taking into account the fact that drugs should be recognized by influx transporters, while efflux transporters at the iBRB be avoided (Hosoya et al. 2009). To effectively implement the current drug delivery strategies, it is critical to understand the distribution and functional importance of the various transporters which are exerting the barrier properties of the eye. Also the varied expression of the influx and efflux transporters in disease conditions needs careful consideration during design of drug delivery systems (Vadlapatla et al. 2014). It might be the key to successful topical, periocular, intravitreal, and systemic drug delivery to the ocular structures.
19.1.4 Importance of Ocular-Specific Novel Drug Delivery for Overcoming Blood–Ocular Barriers for Providing Optimum Therapeutics
Several experimental studies in in vitro and in vivo models promise the possibility of successful therapeutic effect. However, clinical trials could not show the similar beneficial effect due to subtherapeutic concentrations of the drug in the eye (Velpandian 2010). Figure 19.3 shows the existing problem of the ocular drugs and the potential strategies to overcome it.
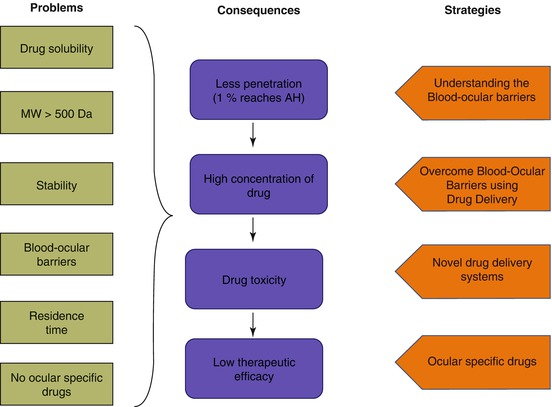
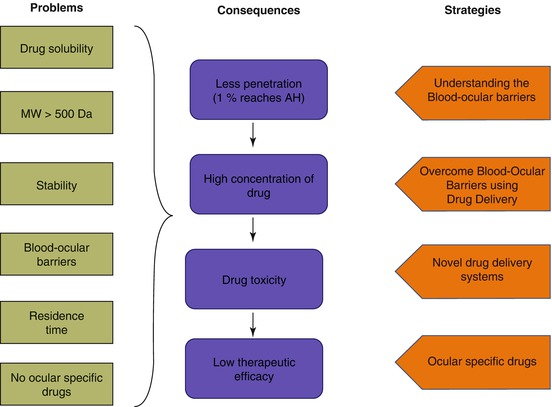
Fig. 19.3
Intricacies with the existing ocular therapeutics
Few of the classical examples discussed below could show the compelling need for the ocular-specific drugs/novel drug delivery systems to overcome blood–ocular barriers and to provide optimum therapeutics for various sight-threatening diseases.
19.1.4.1 Challenges and Potential Opportunities for siRNA Ocular Delivery
Any gene that is responsible for a disease is susceptible to suppression by RNA interference (RNAi) (Guo et al. 2010). Through evolution, it has been a conserved posttranscriptional pathway (Fire et al. 1998). Specific siRNA will be able to target and cleaves the complementary messenger RNA (mRNA), hence inhibits the protein synthesis (gene silencing) through RNAi pathway (Ketting et al. 2001). The synthetically produced siRNA has promising future in the biomedical applications for various diseases. Especially for the eye being a secluded organ, the application of siRNA has great advantage to provide therapeutic activity without unwanted side effects (Guzman-Aranguez et al. 2013). Because of these potential benefits, currently several siRNA therapeutics are being tested at various clinical phase trials by pharmaceutical companies for age-related macular degeneration, diabetic macular edema, and glaucoma (Thakur et al. 2012). However, the clinical studies for bevasiranib and siRNA-027 are terminated or have been completed without significant positive results because of possible failure to meet its key efficacy against inhibiting VEGF or VEGFR1 and possible stimulation of Toll-like receptors 3 (TLR3) (Guzman-Aranguez et al. 2013; Kaiser et al. 2010). Important lessons can be learned from these studies and it is clear that siRNA needs to overcome several barriers to have a successful therapeutic effect. siRNAs electrostatically repel negatively charged cell membrane due to its negative charges from phosphate groups hence cannot cross the membrane and reach cytoplasm. Once it reaches the cytoplasm, it has to escape the endosomes or lysosomes. It also has short half-life in the physiological conditions, poor specificity and tissue uptake, cellular toxicity, and off-target effects (Reischl and Zimmer 2009). Another important issue to consider is that siRNA half-life needs to be at least three times (at the site of action) the half-life of targeted protein for silencing. The rapidly dividing cells and active clearance mechanism of the eye decrease the siRNA half-life hence compels the need for multiple dosing which leads to patient incompliance (Bartlett and Davis 2006).
Drug delivery systems like liposomes, polyplexes, polymers, self-penetrating peptides, and dendrimers could overcome the above said problems to provide optimum siRNA therapeutics in the eye (Thakur et al. 2012). Cells can uptake these nanocarriers through multiple pathways including endocytosis, micropinocytosis, and phagocytosis (Zuhorn et al. 2002). Using these nanocarriers, the siRNA can be delivered to specific cellular compartment and also can escape the endosome/lysosome degradation. For example, polyethylenimine (PEI) is a cationic polymer in which the siRNA could be conjugated (PEI-siRNA delivery system) to deliver sufficient payload inside the cytoplasm through endocytosis and also can escape the endosomes due to its high buffering capability (Boussif et al. 1995).
19.1.4.2 Controversial Results of Aspirin Clinical Trials
Aspirin’s anti-inflammatory property and its effect on DR already has been studied in clinical trials and animal studies. However, prospective clinical trials in humans showed contradictory conclusions, with one study showing a significantly lower mean yearly increase in the number of definite microaneurysms in the aspirin-treated group (DAMAD Study Group 1989), and the other shows no benefit (or harm) of aspirin on the retinopathy (Early Treatment Diabetic Retinopathy Research Group 1991). The aspirin’s failure to inhibit retinopathy in the Early Treatment Diabetic Retinopathy Research study gives us a clue that the dose of aspirin reached the eye was not high enough to have anti-inflammatory effects. It is important to note that all these studies are carried out using oral aspirin therapy (Tang and Kern 2011). The lesson learned from this study compels the need for the sufficient concentration of aspirin required at retina to treat DR. Recent prospective, randomized study using another NSAID, sulindac shows that the salicylates can inhibit development and progression of DR if anti-inflammatory dose is delivered (Hattori et al. 2007). These clinical studies show the need for the ocular drug delivery systems for aspirin to treat DR. The novel drug delivery systems (e.g., nanoparticles) can deliver aspirin to the vitreous compartment in a sustained manner with offered protection from metabolizing enzymes, avoiding cellular toxicity and specific cell uptake using surface modifications or decorations.
19.1.4.3 Partial Protective Effect of Erigeron breviscapus (vant.) Hand. Mazz in Clinical Trial
Velpandian (2010) described BRB as closed gateways. The inability of the active ingredients to cross closed gates could be a possible reason for the failure of randomized, double-blind, clinical trial while commenting on Chinese traditional herb Erigeron breviscapus (vant.) Hand. Mazz for glaucoma (Velpandian 2010). This commentary also compels the need for the ocular delivery systems to deliver therapeutics to overcome ocular barriers for optimum therapeutics.
19.1.4.4 Limited Success of Calcium Dobesilate
Calcium dobesilate (CDO) was developed more than four decades ago for treating DR and chronic venous insufficiency (Zhang et al. 2015). Recently, a meta-analysis study by Zhang et al. (2015) concluded the efficacy of CDO to treat DR. However, despite its multiple modes of action, clinical studies failed to show the significant efficacy to treat clinically significant macular edema (CSME) (Feghhi et al. 2014; Haritoglou et al. 2009). It gave a mixed signal to use CDO for posterior segment diseases. Considering its multiple actions and hypothesizing the lack of ocular penetration of CDO, our group (Velpandian et al. 2009) developed an ocular-specific eye drops and evaluated its pharmacological efficacy against various cataract models. The study reported sufficient transcorneal penetration after single topical administration of CDO eye drops and also significant effect of CDO in vivo cataract model (Velpandian et al. 2009). Pedro Cuevas et al., even though in a different disease condition, reconfirmed the need for ocular-specific drug delivery by demonstrating the significant effect of CDO eye drop to treat primary pterygium. The report also suggested that the failed efficacy of calcium dobesilate in CSME could be due to its unstable nature and lack of ocular penetration after oral administration (Cuevas et al. 2012).
19.1.5 Conclusion
The major goal in providing optimum therapeutics to the eye is to circumvent the various blood–ocular barriers. Even though new ocular drugs have been developed, still effective concentration could not be reached at site of action due to these ocular barriers. Various drug delivery systems have been developed to increase the ocular bioavailability of ophthalmic drugs after topical instillation. But an ideal topical drug delivery is not yet available. Drugs administered systemically also have poor access to retina and vitreous humor. It is also recognized that drug transporter activity is an essential component for ocular barrier function. Transporter-targeted systemic delivery could be an ideal drug delivery system; however, extensive research is needed to establish the functional importance of these transporters to utilize for the drug delivery systems. Although intravitreal administration overcomes both these barriers, it is also associated with several other problems. Therefore, a smart way of drug delivery is required at this stage to treat various sight-threatening diseases.
19.2 Innovative Drug Delivery Systems
Gaurav K. Jain4
(4)
Department of Pharmaceutics, Faculty of Pharmacy, Jamia Hamdard (Hamdard University), New Delhi, India
19.2.1 Introduction
Ocular drug delivery remains a challenging task due to restrictive barrier functionalities of the eye structure. Structural variation of each layer of ocular tissue can pose a significant barrier following drug administration by any route, i.e., topical, systemic, periocular, and intravitreal. Topical instillation as an eye drop formulation is the most widely preferred noninvasive route of ocular drug administration and accounts for ~90 % of the marketed ophthalmic formulations. Nonetheless, the ocular bioavailability is very low (<5 %) with topical eye drop administration (Khar et al. 2010; Patel et al. 2013; Chaurasia et al. 2015; Reimondez-Troitiño et al. 2015). Static and dynamic ocular barriers impede transport and efficacy of various topically administered medications. Furthermore, it is difficult to achieve therapeutic drug concentration to the back of the eye, viz., vitreous, retina, and choroid, following topical eye drops instillation (Nagarwal et al. 2009; Jain et al. 2012). In addition, physicochemical properties of the drug also have an impact on limiting the ocular drug bioavailability. Tight barriers and layers of alternating polarity make the tear film and cornea an important barrier to most low-molecular-weight hydrophilic and lipophilic drugs. Delivery of high-molecular-weight compounds such as nucleic acids, proteins, peptides, Fab fragments, growth factors, and genetic material, which are gaining increased attention in the ophthalmic field, is also challenging (Khar et al. 2010). Indeed, these compounds are rapidly degraded by extracellular enzymes, and their entry, either by a paracellular or by a transcellular route, is totally restricted. Since most drugs poorly penetrate the cornea, fulminating diseases of the posterior segment are required to be treated with either systemic administration or through intravitreal or periocular injections/implants. Therapy with systemic administration requires large doses due to strong blood–ocular tissue barrier, making it an impractical approach. Though direct injections through intravitreal and periocular route appear to be a promising approach to attain high drug concentrations at the back of the eye, these routes are very invasive requiring skilled administration and are associated with a high degree of risk, such as development of retinal detachment and endophthalmitis (Lee et al. 2010).
Clearly, there is a strong case in favor of formulating ocular delivery systems by focusing on improved ocular bioavailability and extended drug effect in targeted tissues. In particular, the improved ocular bioavailability could be achieved by (i) increasing residence time of carrier on the ocular surface, (ii) enhancing corneal penetration, (iii) prolonged release of drug, and (iv) targeting of drug to intraocular tissues. Advances in development of ocular delivery system is continuing and, with the better understanding of physiology and barriers of the eye, has gathered momentum in recent years. Innovative delivery systems that can efficiently target the diseased ocular tissues generate effective and prolonged drug effective concentrations for treating both anterior and posterior segment disorders are emerging (Fig. 19.4).
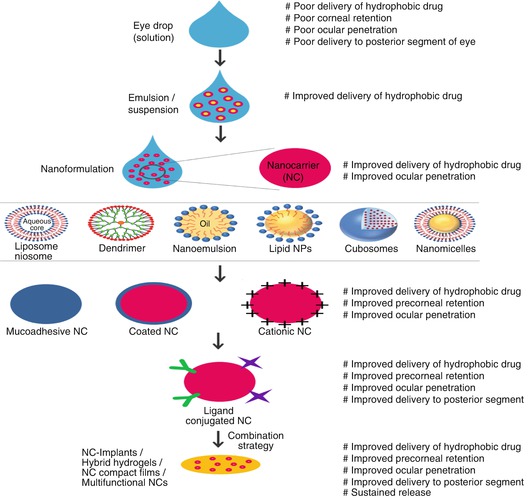
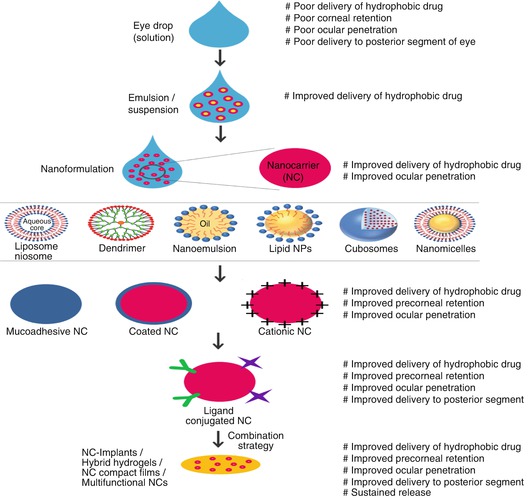
Fig. 19.4
Development stages and attributes of innovative ocular drug delivery systems
Conventional approach to prolong precorneal residence or to improve corneal permeation of topical eye drop formulation is through the addition of viscosity and permeation enhancers. Viscosity enhancers such as hydroxy methyl cellulose, hydroxy ethyl cellulose, sodium carboxymethyl cellulose, and hydroxypropyl methylcellulose improve contact time of drug with the ocular mucosa but suffer from the disadvantage of poor ocular penetration (Patel et al. 2013). Permeation enhancers improve corneal uptake by modifying the corneal integrity. Examples of permeation enhancers investigated for improving ocular delivery include benzalkonium chloride, polyoxyethylene glycol ethers, ethylenediaminetetraacetic acid sodium salt, sodium taurocholate, saponins, and Cremophor EL. However, local toxicity has been observed with the use of permeation enhancers (Patel et al. 2013). To circumvent the problems associated with these delivery systems, emulsions and suspensions have been developed.
These formulations improve both solubility and bioavailability of drug and hold a strong marketplace. Nevertheless, these conventional formulations, because of their micron-size particle range, are associated with stability issues and various side effects such as ocular irritation, redness, inflammation, and vision interference (Patel et al. 2013). In addition, coarse particles do not prevail corneal and conjunctival epithelial barriers of the eye. Attempts to overcome the problems associated with conventional formulations resulted in the development of innovative carriers and technologies that have shown the capacity to (i) encapsulate wide variety of drugs, including biomacromolecules, (ii) reduce the degradation of labile drugs, (iii) increase the precorneal residence time, (iv) improve the corneal penetration, and (v) maintain extended drug effect in targeted tissues. Innovative drug delivery systems such as nanoparticles, nanoplexes, micro- and nanoemulsions, liposomes, niosomes, cubosomes, lipid nanoparticles, nanomicelles, dendrimers, hybrid hydrogels, implants, and drug delivery enhancement devices possess combination of these properties to attain optimum drug levels in desired ocular tissue. Although there are many potential improvements in the field of ocular drug delivery resulting in burgeoning development of innovative ocular drug delivery systems (Fig. 19.4), advancement of these innovative carriers is continuing to allow them to undergo rapid internalization, to enhance target recognition, to sustain the release of encapsulated drug, to enhance gene transfection, and to achieve optimum drug concentration at target site. Such emerging drug delivery technologies have the potential to maintain and/or improve therapeutic indices of active agents. In this section, we provide an overview on the success achieved until now using a number of innovative drug delivery systems for the delivery of drug to ocular tissues.
19.2.2 Innovative Drug Delivery Systems
19.2.2.1 Vesicular Carriers: Liposomes and Niosomes
Vesicular carriers are lamellar structures made up of amphiphilic molecules surrounded by an aqueous compartment. From the early 1980s, vesicular carriers, particularly liposomes and niosomes, have gained wide attention by researchers for their use as ocular drug delivery carriers (Stratford et al. 1983; Kaur et al. 2004). Liposomes are biocompatible and biodegradable vesicles comprising of an aqueous volume surrounded by a phospholipid bilayer (Agarwal et al. 2014). Niosomes are structurally similar to liposomes; however, bilayers are made up of nonionic surfactant instead of phospholipids (Moghassemi and Hadjizadeh 2014). Vesicular carriers are useful for the delivery of both hydrophilic and hydrophobic drugs which are encapsulated in the core or bilayer, respectively. These carriers have been widely explored to overcome the problems of conventional ocular therapy. Vesicles can vary in size from nanometers to micrometers, can be uni- or multilamellar, and can vary considerably in size, surface charge, lipid composition, and fluidity of the bilayers. Drug encapsulation by these carriers results in improved pharmacokinetics, stability, precorneal residence time, and transcorneal permeation. Because of their unique features, liposomes and niosomes are a promising means of delivering ocular drugs for the treatment of both anterior and posterior segment eye disorders. Topical bioavailability can be improved by maximizing corneal drug absorption, minimizing precorneal drug loss, and continuous delivery of ophthalmic drugs to the ocular tissues. When applied topically, vesicles can attach to the hydrophobic corneal epithelium (Schaeffer and Krohn 1982). Effect of vesicle type, charge, and size on precorneal retention and transcorneal permeation is well investigated and generally follows the order of (+)SUV > (+)MLV = (−)SUV > SUV > MLV > free drug. It has been shown that positively charged vesicles composed of cationic lipids such as stearylamine, didodecyldimethylammonium bromide, and 1,2-dioleyl-3-trimethyl ammonium propane have higher binding affinity to the corneal surface than neutral or negatively charged vesicles (Velpandian et al. 1999; Law et al. 2000; Hathout et al. 2007). Several experiments using animal models have shown that cationic liposome-entrapped drugs have improved ocular bioavailability compared to their free drug counterparts. Cationic vesicles also exert adjuvant immunity when they are used as a delivery system for protein vaccines and DNA (Yan et al. 2007). Moreover, the performance of liposomes and niosomes can be further improved by their surface modification. Surface modification of vesicles with carbopol, chitosan, and its derivative, N-trimethyl chitosan, not only improve the physicochemical stability but also prolong precorneal retention and enhance bioavailability over uncoated counterparts and drug solutions (Li et al. 2009; Mehanna et al. 2010; Abdelbary 2011; Li et al. 2012). In a recent study, coating of liposome with mucoadhesive silk fibroin has shown to improve cellular uptake and therapeutic efficacy (Dong et al. 2015). Liposomes incorporated into hydrogels or soft contact lenses or complexed with either chitosan nanoparticle or noisome has been used to improve precorneal retention and to extend optimal ocular pharmacodynamics over a prolonged period (Nagarsenker et al. 1999).
Topical administration for posterior segment delivery is the greatest challenge and opportunity of ocular liposomal drug delivery. It has been shown that liposomal drug can reach the neural retina and ganglion cells after topical administration (Masuda et al. 1996; Davis et al. 2014). Liposome formulation variables might be used to tune the delivery properties to the ocular tissues. It is very unlikely that large-sized liposomes could permeate in the tight corneal barrier. Blood vessel-mediated liposomal delivery to the posterior segment can be achieved only if the liposomes are able to distribute to the ocular tissues. For this purpose, the size of the liposomes is critically important due to the limited size of choroidal and conjunctival fenestrations. Hironaka et al. demonstrated that liposomes with a size of 105–125 nm reach the retina (Hironaka et al. 2009). In another study, size-dependent distribution of liposomes to different posterior segment tissues was seen. Liposomes with the diameter less than 80 nm produced by microfluidization permeated to the retinal pigment epithelium, whereas liposomes with the diameter of 100 nm or more were distributed to the choroidal endothelium (Lajunen et al. 2014). However, active targeting using transferring or phospholipid-binding protein annexin A5 was shown to be necessary for liposome retention to the target tissue (Hironaka et al. 2009; Davis et al. 2014). Direct intravitreal injection of liposomes is a more definitive method of posterior segment drug delivery than topical administration, but it is more invasive and associated with greater risks of infection and bleeding. Despite some advantages that make vesicular carrier a potentially useful system for ocular drug delivery, the utility of these carriers may be limited by a short shelf life, limited drug loading, difficulty associated with thorough sterilization, and long-term side effects. Additionally, vesicles may not be able to release the entire payload of active drug relative to a free solution form. Therefore, all these factors should be taken into account while developing vesicular formulation for ophthalmic application.
19.2.2.2 Polymeric Nanoparticles
Nanoparticles (NPs) are colloidal carriers with a size range of 10–1000 nm. For ocular delivery, nanoparticles are generally composed of proteins, natural or synthetic polymers such as gelatin, modified starch, albumin, sodium alginate, chitosan, hyaluronic acid, carbopol, poly (lactic-co-glycolic acid) (PLGA), poly-ε-caprolactone (PCL), Eudragit® (RS100 and RL100), and poly-(butylcyanoacrylate) (PBCA). Drug-loaded NPs can be nanospheres or nanocapsules. In nanospheres, drug is uniformly distributed throughout polymeric matrix, while in nanocapsules, drug is enclosed inside the polymeric shell. NPs were considered to offer the possibility of a more facile delivery and transport across tissues, and consequently their potential for delivery to both anterior and posterior ocular tissues started to be studied (Khar et al. 2010; Jain et al. 2012). In 1986, an early attempt to use NPs was done with PBCA NPs loaded with highly lipophilic drug, progesterone (Li et al. 1986). After topical application to rabbit eyes, a decreased progesterone concentration in tissues, compared to control solutions, was observed attributed to greater affinity of progesterone for the NP polymer. The lesson learned from these experiments was that it is important to optimize the physicochemical relations between the polymers and drug to obtain an efficient carrier. Later, Juberias et al., used PECL nanocapsules loaded with 1 % cyclosporine (CsA) in a rat model of corneal transplantation rejection. By using PECL nanocapsules, toxic effects of CsA were avoided along with improved ocular penetration; however, corneal graft rejection was not prevented by the CsA-loaded PECL NPs (Juberías et al. 1998). The failure was not considered to be a consequence of negative interactions between the polymer and the drug. Improved formulations using polymers with known biocompatibility and biodegradability, such as PLGA, were developed later and shown to be more effective than commercial formulations (Vega et al. 2006; Garg et al. 2014). Recently, NPs with mucoadhesive properties have been developed to improve precorneal residence time. NPs prepared from chitosan, hyaluronic acid, carbopol, and sodium alginate are commonly employed to improve precorneal residence time of nanoparticles (Kao et al. 2006; Motwani et al. 2008; Ibrahim et al. 2010; Contreras-Ruiz et al. 2010; Ameeduzzafar et al. 2014; Chhonker et al. 2015).
Out of these, chitosan NPs or chitosan-coated NPs are most widely explored for improving precorneal residence. Chitosan is a natural polysaccharide with interesting features, such as biocompatibility and biodegradability, mucoadhesiveness, and the ability to transiently enhance the permeability of ocular barriers. Chitosan NPs are able to interact with the ocular surface and get through the corneal and conjunctival epithelium without causing toxicity. Several studies using chitosan NPs, chitosan/carbopol NPs, chitosan/lecithin NPs, and chitosan/alginate NPs have demonstrated improved pharmacokinetics and pharmacodynamic activity of encapsulated drugs (Motwani et al. 2008). The surface characteristics of the nanocarriers also have an influence on the interaction with the ocular surface structures. For instance, chitosan-coated PECL NPs enter the corneal epithelium in vivo more efficiently than uncoated PECL or polyethylene glycol-coated PECL NPs (de Campos et al. 2003). The transport pathways by which NPs penetrate the ocular surface tissues are of great interest. For NPs composed of hydrophobic polymers such as PECL or PLGA, uptake via transcellular route has been proposed, whereas for NPs composed of hydrophilic polymers such as chitosan, paracellular route has been proposed. These results moved us to explore different biomaterial combinations intended specifically for the ocular surface tissues. Consequently, we joined PLGA and chitosan to form a new matrix-based nanosystem that we termed “nanoplex” (Jain et al. 2010a, b, 2011; Warsi et al. 2014). The theoretical advantages of these nanoplexes are encapsulation of both hydrophilic and hydrophobic drugs, better control over drug release, prolonged corneal retention, and penetration (Jain et al. 2010a, b). We tested nanoplex formulation, showing their potential for ocular administration. In an in vivo study using rabbit eye, we investigated the interaction of nanoplexes with ocular mucosa. Confocal microscopy of the cornea revealed both paracellular and transcellular uptake of the nanoplex. The uptake mechanism postulated was adsorptive-mediated endocytosis and opening of tight junctions between epithelial cells. Results demonstrated prolonged precorneal retention of nanoplexes. Further, these carriers were found to be well tolerated (Jain et al. 2011).
NPs have also been lucratively employed as an alternative strategy for prolonged drug delivery to the posterior segment ocular tissues. For posterior segment delivery, disposition of NPs depends on the size and surface property. Following periocular administration into Sprague–Dawley rats, NPs in the range of 200–2000 nm were retained at the site of administration for at least 2 months. On the other hand, NPs with size 20 nm were cleared rapidly from periocular tissues (Amrite and Kompella 2005; Amrite et al. 2008). Therefore, it can be concluded that for transscleral drug delivery to the back of the eye, NPs with low clearance by blood and lymphatic circulations and slow drug release are suitable delivery carriers. Following intravitreal injection, NPs migrate through the retinal layers and tend to accumulate in the RPE cells. Following single intravitreal injection, the PLA NPs were retained in rat RPE tissues up to 4 months, suggesting great potential for achieving steady and continuous delivery to the back of the eye using NPs. In another study using PLGA NPs, authors concluded that NPs provide sustained delivery of dexamethasone for the treatment of posterior segment eye diseases (Pan et al. 2015). The surface property of nanoparticles is a key factor affecting their distribution from vitreous humor to retinal layers following intravitreal injection. Koo et al. found that polyethyleneimine/glycol chitosan NPs and human serum albumin/glycol chitosan NPs easily penetrated the vitreal barrier and reached at the inner limiting membrane but could not penetrate to the deeper retinal layers. On the other hand, negatively charged human serum albumin/hyaluronic acid NPs could penetrate the whole retina structures and reach the outer retinal layers such as the photoreceptor layer and RPE which was attributed to interaction between anionic surface and Müller cells (Koo et al. 2012). Therefore, the anionic NPs could be promising drug delivery carrier for the treatment of choroid and retinal disorders. Additionally, molecules such as folate and hyaluronic acid have been proposed as a targeting strategy. Specifically, folate-decorated NPs have shown increased uptake in retinal pigment epithelium cells in vitro (Suen et al. 2013). On the other side, the inclusion of hyaluronan into chitosan NPs was explored as a strategy to target the CD44 receptors expressed by epithelial corneal and conjunctival cells (Contreras-Ruiz et al. 2011). Few other reports highlighted the importance of polymeric NPs to deliver drug and genes to the retina more specifically to the RPE cells (de la Fuente et al. 2008; Khar et al. 2010). More recently, novel pentablock copolymeric NPs have been developed using biodegradable polyglycolic acid, polyethylene glycol, polylactic acid, and polycaprolactone polymers. Results indicated that pentablock polymeric NPs can serve a platform for sustained delivery of therapeutic proteins in the treatment of posterior segment diseases (Patel et al. 2014).
19.2.2.3 Dendrimers
Dendrimers are globular, nanostructured polymers (~1–100 nm), which have received significant attention as ocular drug delivery systems, due to their well-defined size, tailorable structure, and potentially favorable ocular biodistribution. Dendrimers have tree-like branched architecture formed by repetitive branched molecules surrounding a central core allowing them to act as efficient carriers for nucleic acids and water insoluble drugs. Because of their electrophoretic, dimensional length scaling and other biomimetic properties, similar to globular proteins, dendrimers display good ocular tolerance. The large number of surface functional groups on dendrimers can lead to multivalent interactions, with a potential for mucoadhesive properties that could lead to a reduction in tear washing and dilution and improved precorneal residence. The most commonly used dendrimers in ocular delivery are poly-(amidoamine) (PAMAM), polypropylenimines (PPI), and phosphorus dendrimers (Yavuz et al. 2013). Although the mechanisms of interaction of the dendrimers with the ocular mucosa have not been fully disclosed, it has been reported that anionic high-generation PAMAM dendrimers can act as permeability enhancers and they are mainly internalized by caveolin-mediated process, whereas neutral and cationic low-generation dendrimers promote higher permeability and are endocytosed by clathrin-mediated pathway (Perumal et al. 2008; Spataro et al. 2010; Durairaj et al. 2010; Kambhampati and Kannan 2013). Various research groups have shown enhanced bioavailability of pilocarpine (Vandamme and Brobeck 2005), tropicamide (Vandamme and Brobeck 2005), puerarin (Yao et al. 2010), brimonidine (Holden et al. 2012), and timolol maleate (Holden et al. 2012) after topical application of PAMAM dendrimer formulation. Phosphorus dendrimers are reported to be beneficial in the delivery of carteolol to aqueous humor (Spataro et al. 2010). Dendrimer glucosamine and dendrimer glucosamine 6-sulfate conjugates have shown to have synergistic immunomodulatory and antiangiogenic effect (Shaunak et al. 2004).
< div class='tao-gold-member'>
Only gold members can continue reading. Log In or Register a > to continue
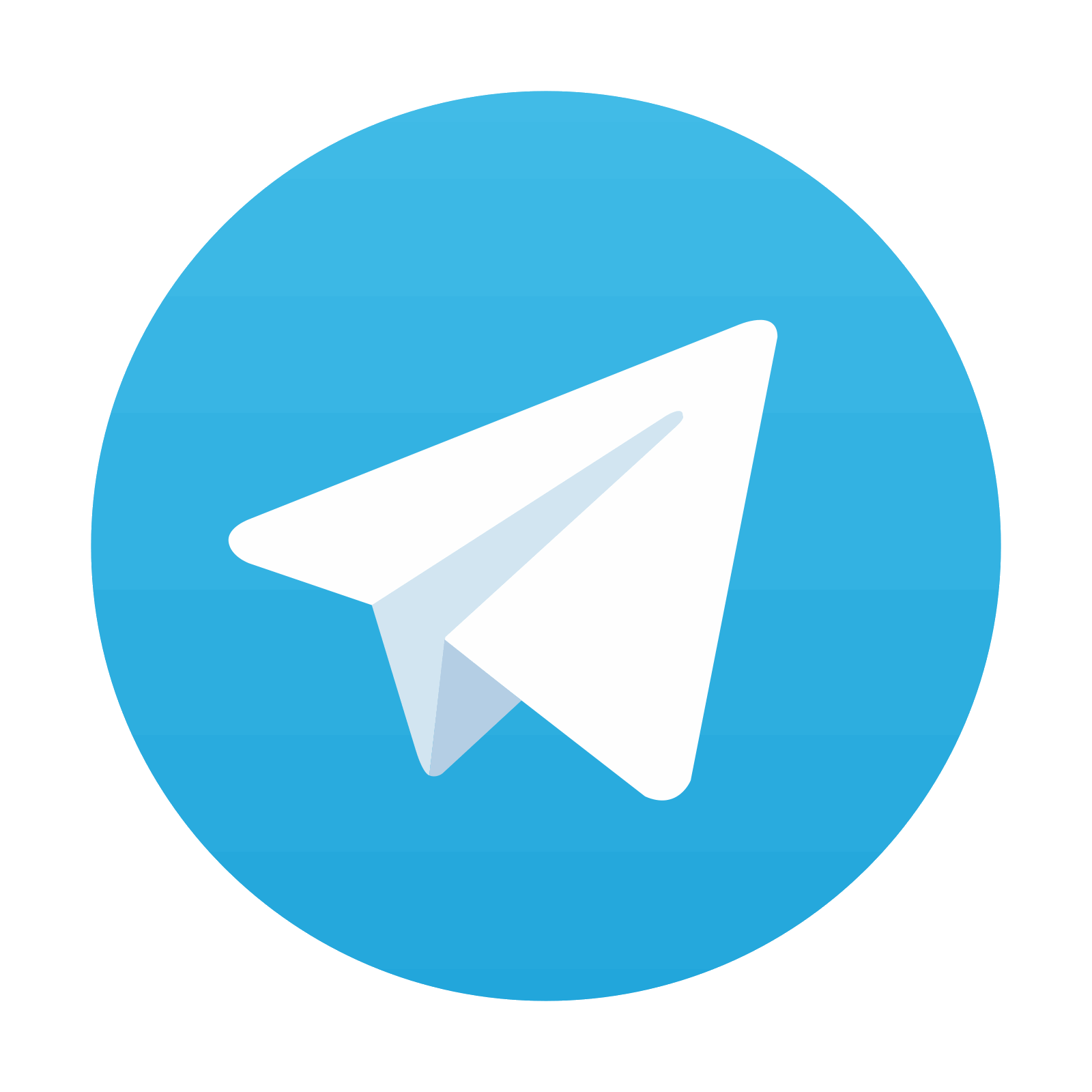
Stay updated, free articles. Join our Telegram channel
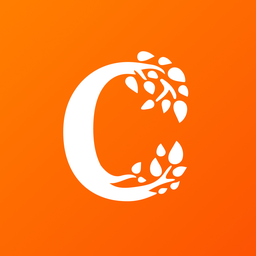
Full access? Get Clinical Tree
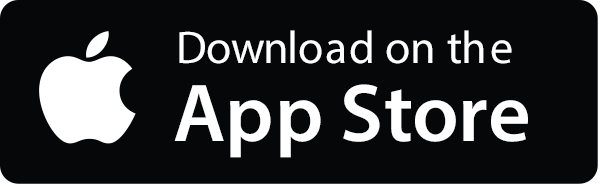
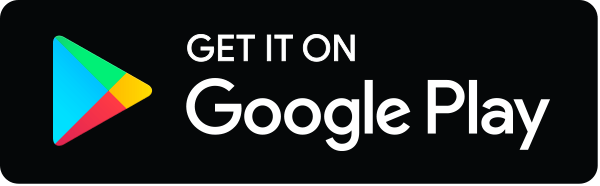