54
The Biochemistry of Aging
OBJECTIVES
After studying this chapter, you should be able to:
Describe the essential features of wear and tear theories of aging.
List at least four common environmental factors known to damage biological macromolecules such as proteins and DNA.
Describe why nucleotide bases are especially vulnerable to damage.
Describe the most physiologically important difference between mitochondrial and nuclear genomes.
Describe the oxidative theory of aging and name the primary sources of reactive oxygen species (ROS) in humans.
Describe three mechanisms by which cells prevent or repair damage inflicted by ROS.
Describe the basic tenets of metabolic theories of aging.
Describe the mechanism of the telomere “countdown clock.”
Describe our current understanding of the genetic contribution to aging.
Explain the evolutionary implications of a genetically encoded lifespan.
BIOMEDICAL IMPORTANCE
Consider the various stages in the lifespan of Homo sapiens. Infancy and childhood are characterized by continual growth in height and body mass. Basic motor and intellectual skills develop: walking, language, etc. Infancy and childhood also represent a period of vulnerability wherein a youngster is dependent upon adults for water, food, shelter, protection, and instruction. Adolescence witnesses a final burst of growth in the body’s skeletal framework. More importantly, a series of dramatic developmental changes occur—an accumulation of muscle mass, loss of residual “baby fat,” maturation of the gonads and brain tissue, and the emergence of secondary sex characteristics—that transform the dependent child into a strong, independent, and reproductively capable adult. Adulthood, the longest stage, is a period devoid of dramatic physical growth or developmental change. With the notable exception of pregnancy in females, it is not unusual for adults to maintain the same body weight, overall appearance, and general level of activity for two or three decades.
Barring fatal illness or injury, the onset of the final stage of life, old age, is signaled by a resurgence of physical and physiological change. Hair begins to noticeably thin, turning white or gray as it loses its pigmentation. Skin loses its suppleness and accumulates blemishes. Individuals appear to shrink as both muscle and bone mass are progressively lost. Attention span and recall decline. Eventually, inevitably, life itself comes to an end as one or more essential bodily functions ceases to operate.
Understanding the underlying causes and instigating triggers of aging and the changes that accompany it is of great biomedical importance. Hutchison-Gilford, Werner’s, and Down syndrome are three human genetic diseases whose pathologies include an acceleration of many of the physiological events associated with aging. Slowing or stopping some of the degenerative processes that cause or accompany aging can render the last stage of life much more vital, productive, and fulfilling. Co-opting the factors responsible for triggering cell death may enable physicians to selectively destroy harmful tissues and cells such as tumors, polyps, and cysts without collateral damage to healthy tissues.
LIFESPAN VERSUS LONGEVITY
From Paleolithic times through Greece’s Golden Age to Medieval times the average life expectancy for a newborn baby remained relatively constant, oscillating within the range of 25-35 years. Beginning with the Renaissance, this number has gradually increased, so that by the beginning of the twentieth century the average life expectancy of persons born in developing countries reached the mid-40s. Today, 100 years later, the current world average is 67 years, and that for developed nations is approaching 80. This has led to speculation in the popular press about how long this trend might be expected to continue. Can future generations expect to live past the century mark? Is it possible that human beings possess the potential, barring accidents and with proper care and maintenance, to live indefinitely?
Unfortunately, this extrapolation is unlikely to be realized because it is based on a misunderstanding of the term life expectancy. Life expectancy is calculated by averaging over all births. Hence, it is dramatically influenced by infant mortality rates. While the life expectancy of a Roman child was 25 years, if one calculated the expected lifespan only for those persons who survived infancy, which we will refer to as longevity, the average nearly doubled to 48. When one factors out the dramatic decline in infant mortality rates that has taken place over the past century and a half, the apparent doubling in the human lifespan largely, but not entirely, disappears. As can be seen in Table 54-1. the predicted longevity of a 5-year-old child in the United States has increased from 70.5 in 1950 to 77.5 years in 2000. Is there some sort of upper limit to the lifespan of a properly nourished, well-maintained human being? Perhaps not.
TABLE 54–1 Average Life Expectancy by Decade, USA
AGING & MORTALITY: NONSPECIFIC OR PROGRAMMED PROCESSES?
Are aging and death nondeterminant or stochastic processes in which living creatures inevitably reach a tipping point where they succumb to a lifetime’s accumulation of damage from disease, injury, and simple wear and tear? While the human body has a certain capacity to repair and replace at the molecular and cellular levels, this capacity is variable and finite. No matter how much attention is devoted to care and maintenance, like an automobile or some other sophisticated mechanical device, sooner or later some key component of our bodies will wear out. An alternative school of thought posits that aging and death are genetically programmed processes analogous to puberty, which have evolved through a process of natural selection.
Aging and death are, in all likelihood, multifactorial processes to which numerous factors, some nondeterminant and others programmed, make important contributions. While much work remains to be done before the precise makeup of this mechanistic mosaic can be determined, a large range of potential contributors have been identified. Several of the more prominent of these are presented in the sections that follow.
WEAR & TEAR THEORIES OF AGING
Many theories regarding aging and mortality hypothesize that the human body eventually succumbs to the accumulation of damage over time due to a variety of environmental factors that are reactive with organic biomolecules. These theories note that while repair and turnover mechanisms exist to restore or replace many classes of damaged molecules, these mechanisms are not absolutely perfect. Hence, some damage leaks through—damage that will inevitably accumulate over time, particularly in long-lived cell populations that experience little, if any, turnover (Table 54-2). Ironically, many of the agents that are most damaging to proteins, DNA, and other biomolecules are also essential for terrestrial life: water, oxygen, and sunlight.
TABLE 54–2 Time Required for All of The Average Cells of this Type to be Replaced
Hydrolytic Reactions Can Damage Proteins and Nucleotides
Water is a relatively weak nucleophile. However, because of its ubiquitous presence and high concentration (>55 M, see Chapter 2), even this weak nucleophile will react with susceptible targets inside the cell. In proteins, hydrolysis of peptide bonds leads to cleavage of the polypeptide chain. The amide bonds most frequently targeted by water are those found on the side chains of the amino acids asparagine and glutamine, presumably because they are more exposed, on average, to solvent than the amide bonds in the protein’s backbone. Hydrolysis leads to the replacement of the neutral amide group with an acidic carboxylic acid group, forming aspartate and glutamate, respectively (Figure 54–1, parts A and B). This change leads to the introduction of both a negative charge and of a potential proton donor or acceptor to the affected region of the protein. As the protein population within a living organism is subject to continual turnover, in most cases the chemically modified protein will be degraded and replaced by a newly synthesized protein.
FIGURE 54–1 Examples of hydrolytic damage to biological macromolecules. Shown are a few of the ways in which water can react with and chemically alter proteins and DNA: (A) Net substitution of aspartic acid via hydrolytic deamidation of the neutral side chain of asparagine. (B) Net substitution of glutamic acid via hydrolytic deamidation of the neutral side chain of glutamine. (C) Net mutation of cytosine to uracil by water. (D) Formation of an abasic site in DNA via hydrolytic cleavage of a ribose-base bond.
Of perhaps greater potential biological consequence are the reactions of the nucleotide bases in DNA with water. The amino groups projecting from the heterocyclic aromatic rings of the nucleotide bases cytosine, adenine, and guanine are each susceptible to hydrolytic attack in which the amino group is replaced by a carbonyl to form uracil, hypoxanthine, and xanthine, respectively (Figure 54–1, part C). If the affected base is located in the cell’s DNA, the net result is a mutation that, if left unrepaired, can potentially perturb gene expression or produce a dysfunctional gene product. The bond between the nucleotide base and the deoxyribose moiety in DNA is also vulnerable to hydrolysis. In this instance the base is completely eliminated, leaving a gap in the sequence (Figure 54–1, part D) which, if left unrepaired, can lead to either a substitution or a frame-shift mutation (see Chapter 37).
Many other bonds within biological macromolecules also possess the potential to be cleaved by random chemical hydrolysis. Included in this list are the ester bonds that bind fatty acids to their cognate glycerolipids, the glucosidic bonds that link the monosaccharide units of carbohydrates, and the phosphodiester bonds that hold polynucleotides together and link the head groups of phospholipids to their diacylglycerol partners. However, these reactions appear to take place too infrequently (phosphodiester hydrolysis) or to generate insufficiently perturbing products to manifest significant biological consequences.
Respiration Generates Reactive Oxygen Species
Numerous biological processes require enzyme-catalyzed oxidation of organic molecules by molecular oxygen (O2). These processes include the hydroxylation of proline and lysine side chains in collagen (Chapter 5), the detoxification of xenobiotics by cytochrome P450 (Chapter 53), the degradation of purine nucleotides to uric acid (Chapter 33), the reoxidation of the prosthetic groups in the flavin-containing enzymes that catalyze oxidative decarboxylation (eg, the pyruvate dehydrogenase complex, Chapter 18) and other redox reactions (eg, amino acid oxidases, Chapter 28), and the generation of the chemiosmotic gradient in mitochondria by the electron transport chain (Chapter 13). Redox enzymes frequently employ prosthetic groups such as flavin nucleotides, iron-sulfur centers, or heme-bound metal ions (Chapters 12 and 13) to assist in the difficult task of generating and stabilizing the highly reactive free radical and oxyanion intermediates formed during catalysis. The electron transport chain employs specialized carriers such as ubiquinone and cytochromes to safely transport single, unpaired electrons among and within its various multiprotein complexes.
Occasionally, these highly reactive intermediates escape into the cell in the form of ROS such as superoxide and hydrogen peroxide (Figure 54–2, part A). By virtue of its structural and functional complexity and extremely high level of electron flux, “leakage” from the electron transport chain constitutes by far and away the major source of ROS in most mammalian cells. In addition, many mammalian cells synthesize and release the second messenger nitric oxide (NO.), which contains an unpaired electron, to promote vasodilation and muscle relaxation in the cardiovascular system (Chapter 49).
FIGURE 54–2 Reactive oxygen species (ROS) are toxic byproducts of life in an aerobic environment. (A) Many types of ROS are encountered in living cells. (B) Generation of hydroxyl radical via the Fenton reaction. (C) Generation of hydroxyl radical by the Haber-Weiss reaction.
Reactive Oxygen Species Are Chemically Prolific
The extremely high reactivity of ROS makes them extremely dangerous. ROS can react with and chemically alter virtually any organic compound, including proteins, nucleic acids, and lipids. In some cases, reaction leads to the cleavage of covalent bonds. They also display a strong tendency to form adducts, the products of the direct addition of two (or more) compounds, with nucleotide bases, polyunsaturated fatty acids, and other biological compounds possessing multiple double bonds (Figure 54–3). Adducts formed with nucleotide bases can be especially dangerous because of their potential, if left uncorrected, to cause misreads that introduce mutations into DNA.
FIGURE 54–3 ROS react directly and indirectly with a wide range of biological molecules. (A) Peroxidation of unsaturated lipids generates reactive products such as malondialdehyde and 4-hydroxynonenal. (B) Guanine can be directly oxidized by ROS to produce 8-oxoguanine or form an adduct, M1dG, with the ROS product malondialdehyde. (C) Common reactions of proteins with ROS, including oxidation of amino acid side chains and cleavage of peptide bonds. Oxygen atoms derived from ROS are marked in red. Carbon atoms derived from malondialdehyde in M1dG are colored blue. The complete chemical name for M1dG is 3-(2-Deoxy-D-erythro-pentofuranosyl)pyrimido(1,2-α)purin-10(3H)-one.
The ease with which oxygen evokes the chemical changes that turn household butter rancid is a testament to the reactivity of unsaturated fats, those containing one or more carbon-carbon double bond (Chapter 23
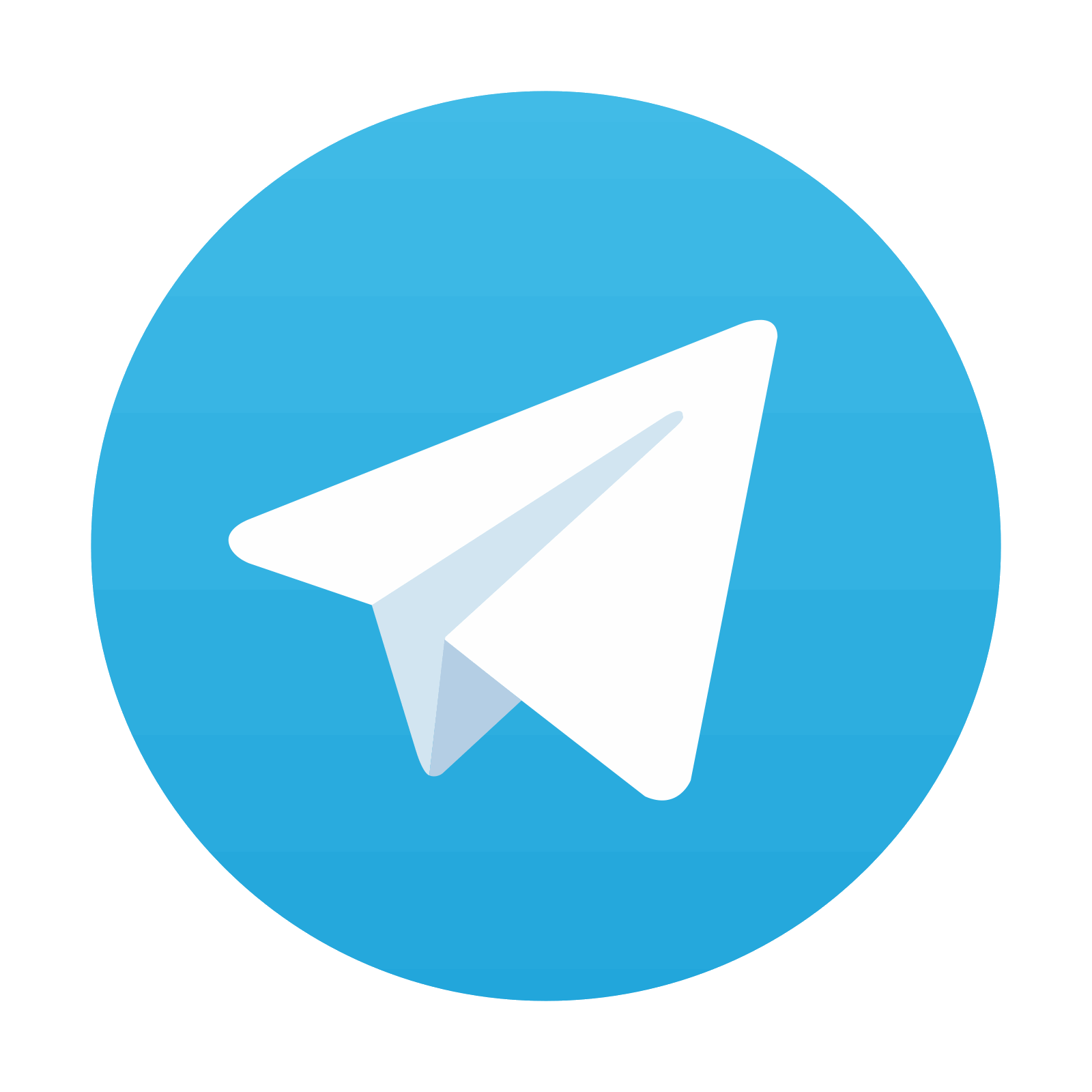
Stay updated, free articles. Join our Telegram channel
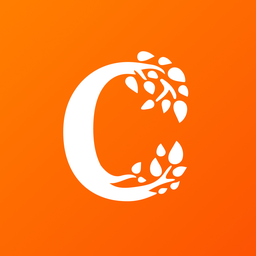
Full access? Get Clinical Tree
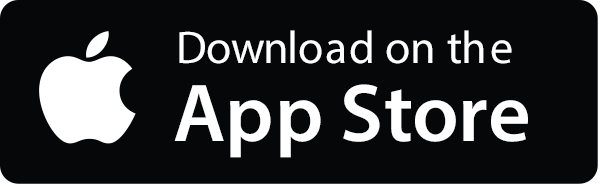
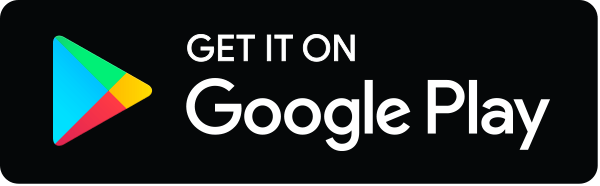