In this chapter we have discussed a wide variety of intermolecular interactions that can play a role in the binding of a drug to its target. In this case study we will discuss a new class of oral anticoagulants approved in the past decade for the treatment and prevention of blood clots and stroke. Examples of this new class include rivaroxaban (Xarelto®), apixaban (Eliquis®), and betrixaban which as of 2014 was still in clinical trials (Figure 2.13). These compounds inhibit the coagulation factor Xa (fXa), which lies at the intersection of signaling pathways common to both the intrinsic and extrinsic branches of the coagulation cascade.
Figure 2.13 Structures of factor Xa inhibitors rivaroxaban, apixaban, and betrixaban.
Factor Xa is a serine protease that converts prothrombin to thrombin, ultimately leading to the formation of a blood clot. Both prothrombin and thrombin are proteases, enzymes designed to break one or more peptide (amide) bonds in their protein substrates (Chapter 6 will include a more extensive discussion of proteases and their enzymatic mechanisms). To carry out the bond breaking hydrolysis reaction at the appropriate site, a protease must first “recognize” a specific amino acid sequence in the substrate. Typically, a substrate protein will bind to the protease with specific amino acid side chains bound in complementary binding pockets in the protease active site. These various side chain binding sites are named by convention S3…S2…S1…cleavage site…S1’…S2’…S3’ etc., where the peptide bond to be broken is situated between the S1 and S1’ sites. Protease inhibitors are usually designed to mimic the interactions formed between the protease and its normal substrate.
The active site of fXa features a box-shaped S4 pocket formed by three aromatic side chains (Figure 2.14, in cyan). This pocket is an excellent example of a poorly solvated protein surface. In most fXa inhibitors then, filling of the S4 pocket contributes significantly to the overall free energy of binding. The S4 pocket is filled by a morpholinone ring in rivaroxaban that expels water molecules while also forming favorable C–H-π interactions with the surrounding aromatic side chains of Tyr99, Trp215, and Phe174. The electron-withdrawing carbonyl group in the morpholinone ring polarizes the neighboring C–H bonds, making them more effective C–H donors. Indeed, a close analog of rivaroxaban lacking the carbonyl group was found to bind fXa about 50 times more weakly (Figure 2.14)!
Figure 2.14 (a) Binding mode of rivaroxaban (shown in green) to factor Xa as determined by X-ray crystallography. Key aromatic side chains of the S4 pocket of fXa are shown in cyan (Tyr99, Trp215, Phe174) while a key aromatic side chain in the S1 pocket (Tyr228) is colored magenta. (b) Relatively small changes to the structure of rivaroxaban lead to significant loss of activity, for the reasons discussed in the text.
The S1 pocket of factor Xa is another important binding subsite for most fXa inhibitors. Important features of the S1 pocket include the aromatic side chain of Tyr 228 and an anionic side chain (Asp189) that binds an Arg side chain in the prothrombin protein substrate. Several early fXa inhibitors were designed to form an ionic interaction with Asp189 and thus had cationic functionality in their structures. While such charged compounds were indeed potent inhibitors of fXa, they often had poor oral bioavailability. This problem was avoided in rivaroxaban by eliminating the ionic interaction with Asp189 and instead exploiting a halogen bond between a chlorine atom on the thiophene ring and Tyr228 in fXa (Figure 2.14).
The morpholinone and chlorothiophene rings of rivaroxaban are connected by an aryloxazolidinone ring system that forms hydrogen bonding contacts within the fXa active site (Figure 2.14, yellow hashed lines). The carbonyl of the oxazolidinone ring accepts a hydrogen bond from the backbone NH bond of Gly219 while the thiophene amide donates a hydrogen bond to the backbone carbonyl of Gly219. These intermolecular interactions of rivaroxaban with fXa are highly cooperative—the hydrogen bonding interactions anchor the core of the inhibitor in a way that allows the thiophene and morpholinone rings to project into the S1 and S4 pockets, respectively. When the amide connection to the thiophene ring was replaced with a sulfonamide or N–Me amide, a ~300-fold loss of potency resulted (Figure 2.14). Changes this dramatic are unlikely to result from the loss of a single hydrogen bond. More likely, the change to a sulfonamide or N–Me amide negatively affects binding cooperativity by less optimally positioning the morpholinone and chlorothiophene rings in the S4 and S1 binding pockets.
Having discussed the binding of rivaroxaban in some detail, it will be interesting to inspect the structures of the other factor Xa inhibitors presented in Figure 2.13. Can you predict which ring systems in apixaban and betrixaban might bind in the S1 and S4 pockets of fXa? Would the interactions of these other inhibitors at S1 and S4 be similar to rivaroxaban or different in some way? Also, can you predict which atoms in apixaban and betrixaban might accept or donate a hydrogen bond from the backbone amide of Gly219? Do you predict any differences for these inhibitors compared to rivaroxaban? Getting the “right” answers to these questions is less important than the act of generating hypotheses about how these related but different inhibitors interact with their common target.
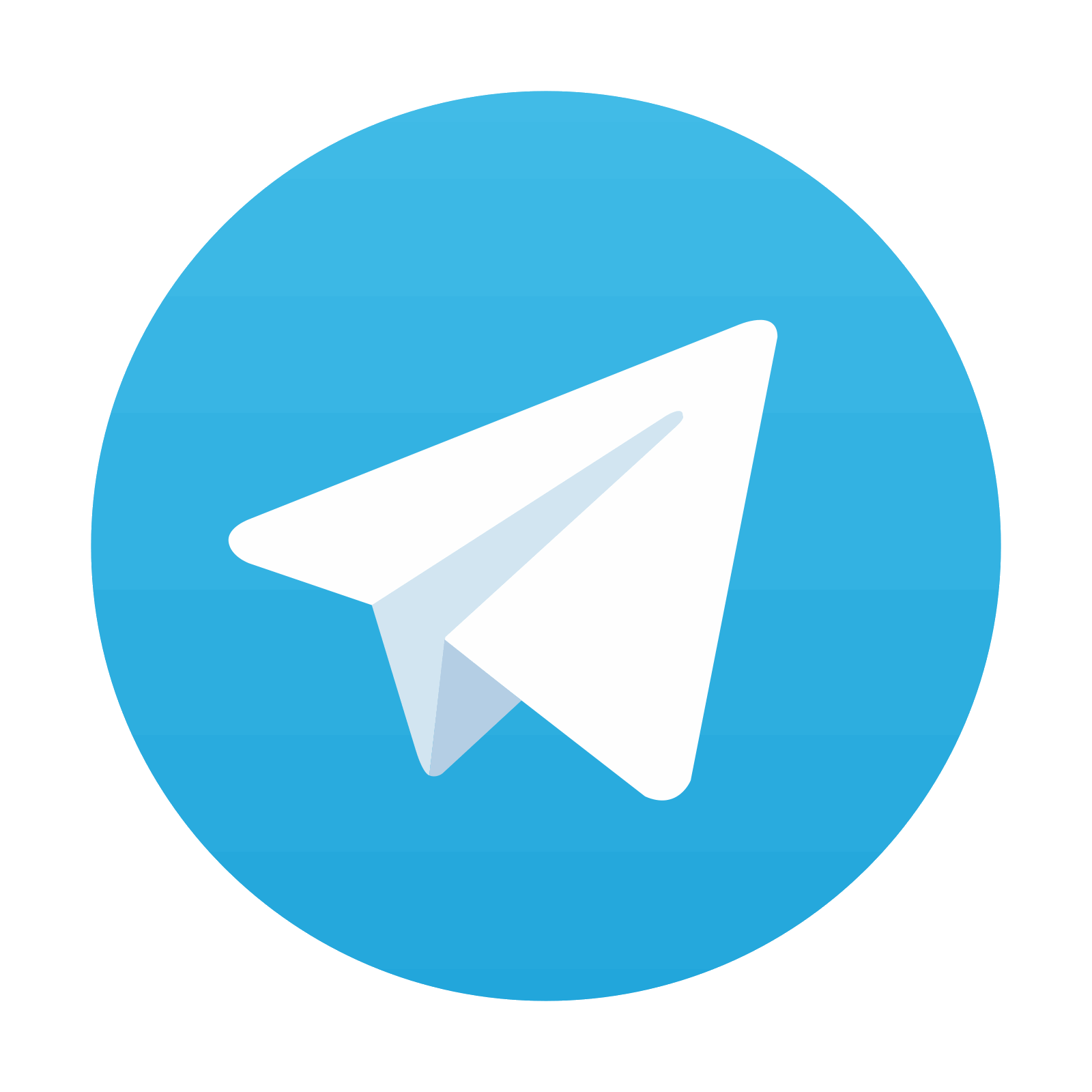
Stay updated, free articles. Join our Telegram channel
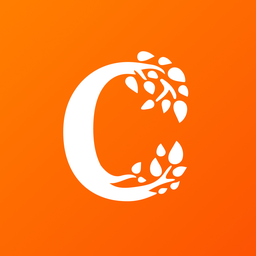
Full access? Get Clinical Tree
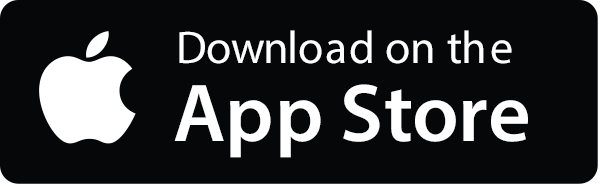
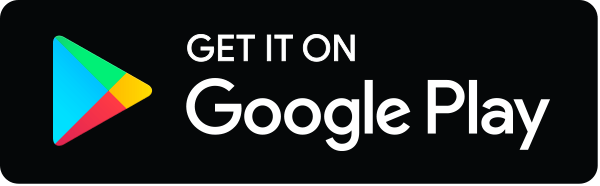