Chapter 1 The structure of the normal lungs
Anatomy of the lungs and airways
The right main bronchus follows more closely the direction of the trachea and, because it branches earlier, is only half the length of the left. The right main bronchus is wider than the left and carries 55% of each breath. It enters the lung behind the right pulmonary artery and is said to be ‘eparterial’ whereas the left main bronchus crosses behind the left pulmonary artery to enter the lung below it and is therefore said to be ‘hyparterial’ (Fig. 1.1).
The two lungs, enclosed within the visceral pleura, fill their respective pleural cavities. Tissue is reduced to a minimum in the interests of gas exchange. At mid-inspiration over 80% of lung volume is air, 10% is blood, 3% comprises conductive airways and blood vessels and only 3% is alveolar tissue. The normal combined weight of the lungs averages 850 g in men and 750 g in women. Lung weights in children are given in Table 1.1.1
The lungs abut the mediastinum medially, rest on the diaphragm below and elsewhere are enclosed by the ribcage. On their medial surface the main bronchovascular structures connect the hilum of the lung with the mediastinum and here the visceral pleura is reflected off the lung to become the parietal pleura. The pleural reflection also extends from the inferior aspect of the hilum to the medial border of the base of the lung at what is termed the pulmonary ligament (see Fig. 1.1B) The pleura is described in more detail in Chapter 13.
The airways of the first four generations are individually named and the pathologist must be familiar with the terminology of the 19 lung segments and their bronchi so that lesions can be localised accurately and the pathological findings correlated with those of radiologists and surgeons. Figure 1.2 illustrates the trachea, main, lobar and segmental bronchi diagrammatically, using an internationally recommended nomenclature.2 A plastic cast of the proximal airways coloured according to the lung segments is shown in Figure 1.3. It will be noted that the following anatomical features are common to the two lungs:
The two lungs differ in the following respects:
Beyond the segmental bronchi, individual airways are unnamed but a distinction is drawn between bronchi, which have glands and cartilage in their wall, and bronchioles, which do not. Transition from bronchus to bronchiole takes place in airways approximately 1 mm in diameter. The first orders of bronchioles, which are known as membranous bronchioles, are purely conductive. The last order of these bronchioles is known as terminal bronchioles, despite them leading into further orders of bronchioles. These further bronchioles have alveoli opening directly off them and are known as respiratory bronchioles; they both conduct gas and participate in gas exchange. Such airways form what is termed a transitional zone between the purely conductive airways and the alveoli. It comprises about three generations of respiratory bronchioles and two to nine generations of alveolar ducts, the last of which terminate in alveolar sacs; all these structures have increasing numbers of alveoli opening off their walls. The subdivisions and structure of the intrapulmonary airways are represented diagrammatically in Figure 1.4 and numerical data appertaining to individual orders (generations) of airways are shown in Table 1.2.3
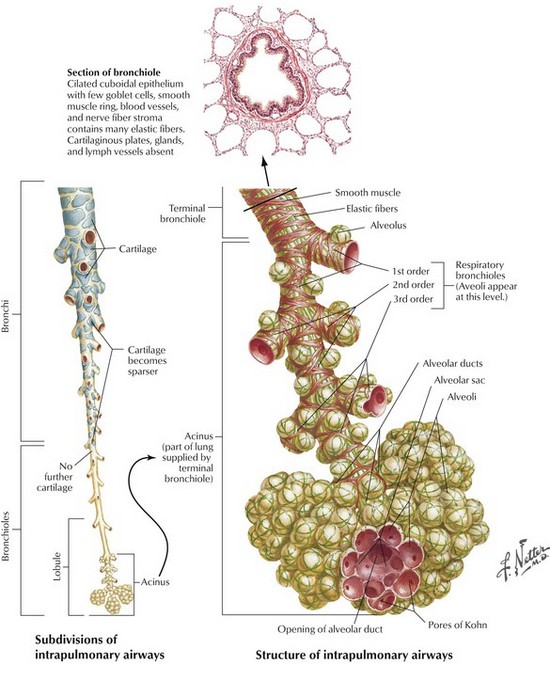
Figure 1.4 Subdivisions and structure of the intrapulmonary airways from a segmental bronchus to the alveoli.
(Netter medical illustration used with permission of Elsevier. All rights reserved.)
The lung substance is divided into primary and secondary lobules. The primary lobule is that portion of the lung supplied by one respiratory bronchiole but it is seldom referred to today and the unqualified term ‘lobule’ generally equates to the secondary lung lobule. The secondary lobule is a portion of lung surrounded by fibrous septa that are visible on the cut surface of the lung (Figs 1.5 and 1.6) and through the visceral pleura, particularly when their contained lymphatics are outlined by dust or tumour (Fig. 1.7). However, the fibrous septa that demarcate the secondary lobules are unevenly developed. They are quite well represented laterally in the lower lobes (where they appear as Kerley B lines when thickened by interstitial oedema) but are poorly represented medially and deep in the lungs. The lobules they demarcate are polyhedral. In the adult lung, each lobule measures 1–2 cm across and is in turn made up of about 3–10 acini, an acinus being that portion of the lung supplied by one terminal bronchiole. The volume of a lobule is approximately 2 ml and that of an acinus approximately 0.2 ml. An acinus is 0.5–1 cm long,4 so that the bronchi and purely conductive bronchioles make up all but this length of the total gas pathway. Each acinus contains about 2000 alveoli and it is estimated that the two lungs together contain about 300 million alveoli.5 The edges of adjacent acini interdigitate without any intervening septa and it is impossible to detect where one acinus ends and the next begins, either with the naked eye or the microscope. However, in adult lungs, the centres of the acini are generally ‘tattooed’ with carbon for it is here that lymphatics commence and dust-laden cells accumulate in what Macklin termed ‘dust sumps’ (see Figs 1.7 and 7.1.12, p. 339).6
The term ‘terminal respiratory unit’ has been applied to an imprecise number of bronchioles and the alveoli they supply, mainly by embryologists and more recently by pathologists and molecular biologists interested in the histogenesis of adenocarcinomas composed of bronchiolar Clara cells and type II alveolar cells (see below and p. 553).7,8 This is because these cells ubiquitously express the regulatory thyroid transcription factor-1 (TTF-1), which is potentially a lineage-specific survival oncogene of some lung adenocarcinomas.9,10
Each generation of airways is shorter than its predecessor and, whereas individual members of each generation are narrower than their parents, beyond the segmental bronchi the summed cross-sectional area for each generation progressively increases logarithmically (Fig. 1.8),3,11 and at the periphery resistance to airflow is negligible. Because of this, gas flow velocity falls rapidly in the respiratory bronchioles and gas mixing in the last few airways is largely by diffusion. Diffusion across tissue is less efficient than in the gas phase but at the surface of the alveolar walls gas transfer is maximised as the inspired gas is spread over an area of about 70 m2.12 There is therefore considerable reserve in the conductive capacity of the peripheral airways, large numbers of which may be obliterated before there is any appreciable shortness of breath; for this reason the finer airways have been termed the ‘silent area of the lung’.
Lambert’s canals are tubular communications approximately 30 µm in diameter that connect terminal and respiratory bronchioles with adjacent peribronchiolar alveoli, thus bypassing the main pathway.13 They represent accessory air inlets to, or outlets from, the more distant alveoli. In coal miners, these canals and their associated alveoli are early sites of dust cell accumulation, whilst in fibrotic lung disease, cuboidal bronchiolar epithelial cells often extend through them to line the peribronchiolar alveoli, a metaplastic process that is termed alveolar bronchiolisation, peribronchiolar metaplasia or lambertosis (Figs 1.9 and 3.37A, p. 121).14
To what extent the 30-µm diameter Lambert’s canals provide collateral circulation is uncertain. They appear to offer a better anatomical pathway than the 2–13-µm diameter pores of Kohn, described below, but may be less effective than certain interconnecting respiratory bronchioles that measure 120 µm in diameter,15 and the 200-µm diameter interacinar and 80–150-µm diameter intersegmental connections that have been demonstrated using injection techniques16 and corrosion casts17 respectively.
The two main pulmonary arteries arise from the bifurcation of the pulmonary trunk shortly after its origin from the right ventricle and divide to follow the lobar bronchi. Segmental and subsegmental pulmonary arteries continue alongside their corresponding airways and in the periphery of the lungs most small pulmonary arteries enter an acinus with the terminal bronchiole and are thus found at the centre of the acinus (Figs 1.10 and 1.11). As well as these axial vessels there are also small supernumerary branches which do not accompany airways.18 The pulmonary arteries divide to supply the pulmonary capillaries, which form a meshwork situated in the alveolar walls, regrouping at the periphery of the acinus to form pulmonary veins (Fig. 1.12). In the periphery of the lung the pulmonary veins run in the interlobular septa and are thus separate from the artery and airway in the centre of the acinus (see Figs 1.6 and 1.10), but they take up a position alongside the artery and bronchus proximal to the entry of these structures into the lung lobule.
Microscopy of the airways
The bronchial wall consists of a thin mucosa and a more substantial submucosal coat, outside which there is the peribronchial sheath of loose connective tissue that also surrounds the accompanying pulmonary artery. The mucosa consists of respiratory epithelium resting on a basement membrane and beneath that a supportive connective tissue layer rich in elastin fibres. There is no clear boundary between the mucosa and the submucosa but muscle, glands and cartilage are conventionally regarded as belonging to the submucosal coat (Fig. 1.13).
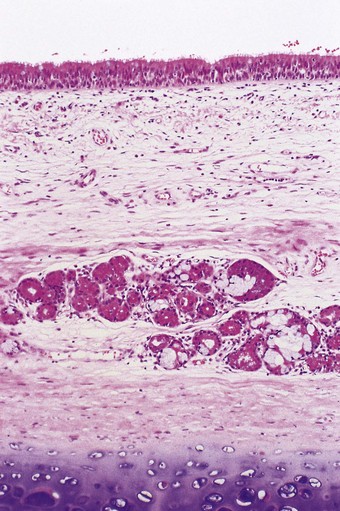
Figure 1.13 Normal bronchus, showing surface epithelium resting on elastin-rich connective tissue (these elements constitute the mucosa: see Fig. 1.14), beneath which are the submucosal glands and cartilage. The glands are of mixed seromucous type. The apparent absence of submucosal muscle at this point is attributable to its double spiral arrangement.
Smooth muscle, present only in the dorsal intercartilaginous gaps of the trachea, completely encircles the intrapulmonary bronchi, internal to the cartilage. It comprises two sets of fibres that wind around the bronchial tree as opposing spirals, thereby appearing incomplete in individual cross-sections. The arrangement is such that, as the muscle contracts, the airway both shortens and constricts. The muscle forms a more complete ring in the membranous bronchioles than in bronchi or respiratory bronchioles. When respiratory bronchioles are encountered in longitudinal section, the muscle is seen only as small knobs between the mouths of the numerous alveoli that open off these airways.
If the trachea and main bronchi are opened from the front, longitudinal mucosal corrugations or ridges are evident on the posterior wall.19 Most of those in the trachea pass into the right main bronchus but there is an equal number in the left main bronchus where they commence anew beyond the carina. In the trachea and main bronchi the ridges are limited to the membranous parts but distal to the lobar bronchi the ridges are distributed all around the walls of the airways. They represent longitudinal bundles of elastin fibres situated in the subepithelial mucosal lamina propria (Fig. 1.14). Although the bundles become progressively thinner they persist throughout the bronchial tree and link up with spiral elastin fibres described in the alveolar ducts20 and with the elastic tissue of the alveolar walls. They are thought to contribute to elastic recoil during expiration and are more prominent in men, older subjects, smokers and asthmatics.21 Transverse ridges are also evident in the bronchial mucosa; these lack elastin fibres and probably represent folds produced by muscular shortening of the airways.
Around the pulmonary arteries and to a lesser extent the airways there is a sheath of loose connective tissue (see Fig. 1.11). This connects with the visceral pleura at the hilum and distally with the delicate connective tissue of the respiratory bronchioles and the alveolar interstitium, and through the latter with the interlobular septa and the visceral pleura. The periarterial sheath carries nerves and lymphatic vessels and is expanded considerably in oedema, acting as an interstitial sump for extravascular fluid and thus protecting against this spilling over into the alveoli.
The epithelium of the main airways is pseudostratified, meaning that all its cells rest on the basement membrane but not all reach the airway lumen (Figs 1.15 and 1.16). Most epithelial cells are columnar and ciliated but these are interspersed with mucous cells, finely granulated neuroendocrine cells, basal cells, brush cells, nerve terminals and migratory lymphocytes and mast cells.22–24 The airway epithelium decreases in height distally, becoming cuboidal in the bronchioles. The mucous cells decrease in number as the bronchioles are approached and in these small airways Clara25 and serous26 cells are found amongst the ciliated cells.27 Major histocompatibility antigens of both class I (HLA-A, B and C) and II (HLA-DR) are expressed by bronchial, bronchiolar and alveolar epithelium.28 All airway epithelial cells express cytokeratin 19 while the basal cells also express cytokeratin 17.29 In contrast to pleural mesothelium, cytokeratins 5 and 6 are not expressed by airway epithelium and rarely by its malignant derivatives, providing a useful point of distinction between pulmonary adenocarcinoma and epithelioid mesotheliomas (see p. 724).30
In the main airways there is a surface layer of mucus supported by an aqueous hypophase (Fig. 1.17). More distally, the bronchial mucous layer is discontinuous.31 In the smaller bronchioles there is a continuous surface layer similar to that lining the alveoli (see below).32
The thickness of the surface layer is of considerable importance. In its proximal movement, the surface layer is constantly being added to by airway secretions yet this material has all to be accommodated on a progressively reducing surface area (see Fig. 1.8). Despite losses through evaporation, and clearance being faster proximally (Fig. 1.18),33 the larger airways would be occluded by the bronchial secretions if these were not concentrated by an epithelial ion exchange mechanism. This vital function is under the control of an epithelial transmembrane regulator, faults in which underlie the development of cystic fibrosis (see p. 65). The same control mechanism operates in reverse in panting dogs to facilitate heat loss. The thickness of the aqueous hypophase is also of crucial importance to ciliary function (see below).
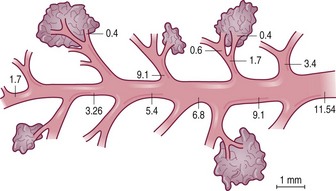
(Reproduced from Iravani & van As (1972)33 by permission of the editor of the Journal of Pathology.)
The various epithelial cells are held together by desmosomes, gap junctions and near the lumen by terminal bars that prevent excessive fluid movement across the epithelium.34 However, irritants such as tobacco smoke, sulphur dioxide and mast cell mediators render the terminal bar permeable to particulate markers placed in the lumen.35–37
Surface epithelial cells are replaced only slowly, less than 1% being in division at any one time, although the mitotic index increases in response to various forms of injury.38 The role of the basal cell as the sole progenitor has been questioned following recognition that other non-ciliated cells, notably the mucous, Clara and neuroendocrine cells, have an important stem cell function.38–45
Approximately 10000 litres of air are inhaled each day and considerable amounts of potentially harmful environmental agents escape the filtering action of the nose to reach the lower respiratory tract. The respiratory epithelium provides the first line of defence against these.46 It does this in three ways:
Nevertheless, immune reactions are constantly at play in the lungs and if these were allowed to proceed unchecked, inflammation would be a permanent feature of the airways. That this is not the case is due at least in part to an inhibitory action that airway epithelial cells exert on dendritic cells within the epithelial milieu.47,48
The morphology and function of the individual epithelial cells will now be considered in turn.
Ciliated cells
Ciliated cells49 possess numerous mitochondria and two types of surface projection: stubby microvilli about 0.4 µm in length and long slender cilia up to 6 µm in length. There are 200–300 cilia per cell, each beating at about 20 times a second. The rate at which the cilia beat falls with temperature50 and the upper respiratory tract plays an important role in warming and moistening the inspired air. The ciliary beat consists of a straight-armed effective stroke followed by a curling return stroke.51 Ciliated cells are arranged in groups within which the cilia beat in a co-ordinated fashion, probably governed by contact with the overlying mucus. The beating appears to be spontaneous. It is independent of nervous control and persists for several hours after death.
The cilia beat in a low viscosity layer beneath the surface mucus and move the overlying mucus only by their tips, which possess minute terminal hooklets (see Fig. 1.17).52 The depth of the aqueous hypophase is crucial to ciliary action: too much and the mucus is lifted off the tips of the cilia, too little and the cilia become clogged by mucus. The periciliary fluid is thought to derive from the airway epithelial cells under the control of the transmembrane regulator referred to above. The cell surface available for fluid transport is greatly increased by its microvilli, which are akin to the brush border of the intestinal epithelium.
The fine structure of cilia has become of medical importance with the recognition of the ciliary dyskinesia syndromes (see p. 63). Cilia have an axial central pair of microtubules with an outer ring of nine double microtubules (9 + 2 structure) (see Fig. 2.44, p. 64). Small dynein side arms extend from one doublet towards the next and spokes connect each doublet with the central microtubules. All the microtubules fuse together near the tip while near the cell the central pair disappears and the doublets become triploid and fuse together as a cylinder which extends into the cytoplasm as the ciliary basal body. Cilia beat when the microtubules, powered by adenosine triphosphate elaborated in the dynein arms, slide past each other.
Basal cells
Basal cells are found particularly in the large airways but, although they diminish in number peripherally, they reach down as far as the bronchioles. They have only sparse cytoplasm, which often contains bundles of cytokeratin tonofilaments that lead into prominent hemidesmosomes.53 The basal cell was formerly thought to be the main stem cell of the airways but with the recognition that Clara, mucous and neuroendocrine cells are important in this respect,38–40,42,45 this view has had to be modified. Although they continue to be recognised as progenitor cells,54 adhesion of the columnar cells to the basement membrane is now thought to be an additional major function of basal cells.55,56
Mucous cells
Mucous cells24,49 vary in appearance with a cycle of secretory activity that culminates in the discharge of mucus into the airways. Devoid of mucus, they are slender and possess abundant endoplasmic reticulum and well-developed Golgi apparatus. As they synthesise mucus, electronlucent mucous granules accumulate, enlarge and fuse together to produce a large secretion vacuole that distends the apical cell cytoplasm, giving the mature cell its characteristic wine glass or goblet shape. Discharge takes place by further fusion involving the secretion vacuole and apical cell membranes.
Mucins are high-molecular-weight glycoproteins in which oligosaccharide side chains are attached to an elongated protein core.57 The mucous granules of the surface mucous cells contain an acidic mucin, with sialic acid or sulphate groups at the end of the oligosaccharide side chains of the peptide core. The amount and viscoelasticity of the mucus are important to airway clearance and the chemical structure of the mucus probably influences its physical properties and hence the ease with which it is cleared by ciliary activity or coughing. Out of the nine different mucin genes identified in human tissues, seven are expressed in the respiratory tract: MUC1–MUC4, MUC5AC, MUC5B and MUC7.58,59 While MUC5B and MUC7 expression is predominantly restricted to cells of the submucosal glands,60 MUC2 and MUC5AC mucins are located more in the surface epithelium.61 The predominant components of respiratory mucus are MUC5AC and MUC5B,62 and these are upregulated by various stimuli such as air pollutants or bacteria and also in asthma and cystic fibrosis.63,64 The number of mucous cells increases in response to irritation. This also induces inflammation and, although the viscosity of mucus is primarily dependent on its glycoprotein content, it is markedly augmented by DNA released from effete inflammatory cells. It is impossible to state at exactly what point goblet cell hyperplasia begins as numbers vary dependent on the site of the biopsy, but a crude figure is more than 3 per 10 cells at any point in the respiratory tract.
Apart from the mucus secreted by mucous cells in the surface epithelium and submucosal glands (see below), non-secretory cells such as the ciliated cells have a thin mucoid glycocalyx forming the outer part of their cell membrane.57 This differs chemically from the main mucous lining and is probably formed by the cell of which it forms the outermost part. The glycocalyx of the cilia differs chemically from that of the microvilli on the same cell, whilst in the alveolus, different lining cells have chemically different forms of glycocalyx.65
Submucosal glands
The submucosal glands far exceed the secretory elements of the surface epithelium in cell mass and are the major source of bronchial secretion. They are situated between the muscle coat and the cartilage (see Fig. 1.13), and between individual cartilage plates, their ducts piercing the muscle coat and mucosa to reach the lumen. They are mixed seromucous glands, and the secretory acini are arranged so that the serous secretion has to pass through the mucous tubules.66 The two secretions are therefore well mixed before they reach the bronchial lumen. Serous and mucous cells both have abundant rough endoplasmic reticulum and Golgi apparatus but the secretory granules of the serous cells are electron-dense, small and discrete, whereas mucous granules are electron-lucent, large and confluent (Fig. 1.19).67 The serous granules often show zonal variations in their fine structure, implying a variety of secretory products. The mucous cells contain large amounts of both acid and neutral glycoprotein whilst the serous cell granules contain smaller amounts of these glycoproteins65,68 together with lysozyme,67 lactoferrin68 and a small-molecular-weight antiprotease specific to the airways.69 The demonstration of carbonic anhydrase in serous cells implicates them in the production of a watery non-viscous secretion that could facilitate transport of the more viscous mucus secreted downstream.66 The serous cells are also the major site of the secretory component of IgA70–72 and of epithelial peroxidase.73
Collecting-duct cells are devoid of secretory granules but often have the characteristics of oncocytes, their cytoplasm being packed with numerous mitochondria. The oncocytes increase in number with age and may form metaplastic nodules (Fig. 1.20).74 A fluid-regulating role has been suggested for them but similar cells present in several other organs are considered to be degenerative. Glandular secretion is assisted by myoepithelial cells which are situated between the secretory and duct lining cells and the basement membrane. Neuroendocrine cells similar to those in the surface epithelium are also found in this situation, whilst unmyelinated nerve axons are observed in close association with serous, mucous, collecting-duct and myoepithelial cells.75
Neuroendocrine cells
Neuroendocrine cells, which are also known as Kultschitsky-type cells, Feyrter cells and APUD cells, are found in the basal layer of the surface epithelium and in the bronchial glands.75,76 In the surface epithelium they occur singly and in groups, the latter known as neuroepithelial bodies.77,78 Single neuroendocrine cells are basally situated but send a thin cytoplasmic process to the surface. They are found throughout the airways from the main bronchi to the bronchioles but are only rarely found in the terminal bronchioles and alveoli.79,80 Neuroepithelial bodies extend from the basement membrane to the surface. They are found particularly at airway bifurcations and have sensory neuronal connections but are less numerous in humans than in many laboratory animals.78,81–85 Adjacent capillaries have a fenestrated endothelium, as found in many endocrine organs.
Neuroendocrine cells are characterised by numerous small granules, 70–150 nm in diameter, consisting of a round electron-dense central core separated from an outer membrane by a clear halo (Fig. 1.21). In the fetus, two other morphological varieties of granule have been described.77 The granules are scattered throughout the cytoplasm, but are often concentrated near the basal cell membrane. The neuroendocrine cells only occasionally display argyrophilia or formaldehyde-induced fluorescence but these reactions, indicative of biogenic amines, may be enhanced by prior incubation with precursor substances such as 5-hydroxytryptophan and dihydroxyphenylalanine.86 Immunohistochemistry shows that both single neuroendocrine cells and the neuroepithelial bodies may contain L-amino acid decarboxylase87 and 5-hydroxytryptamine,88 general neuroendocrine markers such as neuron-specific enolase,89 chromogranin A,80,90 synaptophysin91 and neural cell adhesion molecule (CD56)92 and peptides such as human bombesin (gastrin-releasing peptide),80,93–96 calcitonin,80,94,97,98 leu-encephalin,94 substance P,99 guanine nucleotide-binding protein100 and adrenocorticotrophic hormone.95 Chromogranin, a constituent of the granules, and CD56 are probably the most sensitive and specific immunocytochemical markers.80,92,101,102
The main function of the pulmonary neuroendocrine system in humans is thought to concern the control of growth and development of the lungs in utero and thereafter the regulation of pulmonary regeneration and repair. Neuroendocrine cells are more numerous in the fetal than the adult lung and one of their products, human bombesin, has trophic properties in regard to the other cells. Hyperplasia of neuroendocrine cells has been described during epithelial repair,42,103,104 following asbestos exposure105 and in pulmonary fibrosis,106 infantile bronchopulmonary dysplasia,107 pulmonary arterial disease,108 bronchiectasis associated with tumourlets95 and bronchi bearing carcinomas of all cell types.109 Neuroendocrine hyperplasia plays a role in the development of carcinoids and is thus regarded as a preneoplastic condition.
A subsidiary function of the neuroendocrine cells, which appears to be better developed in lower species, concerns the pulmonary response to hypoxia. Animal experiments have demonstrated that neuroendocrine cells increase in number and degranulate in hypoxic conditions, suggesting a chemoreceptor function.110–113 In the case of the neuroepithelial bodies this function is modulated by intrapulmonary axon reflexes.82 However, the relevance of these observations to humans is uncertain.
Clara cells
These cells are named after the Austrian histologist Max Clara who provided a detailed description of them in 1937.25 They are most numerous in the terminal bronchioles where they protrude above the ciliated cells (Fig. 1.22). The region immediately above the nucleus contains many large mitochondria with unusually sparse cristae. The apical portion is notable for a rich smooth endoplasmic reticulum network and, immediately beneath the luminal cell membrane, small electron-dense secretory granules of about 500 nm diameter. The granules are angulated in humans but round in many species. There is considerable species variation in the internal structure of Clara cells. The nature of the secretory product of the Clara cell and its precise mode of secretion are uncertain, but the major constituent of the granules is a 10-kDa protein (CC10) that inhibits several inflammatory cytokines and is therefore thought to be important in modulating bronchiolar inflammation and protecting the lung against emphysema69; mice that are deficient in this protein are more susceptible to the damaging effects of hyperoxia.114 Cytochrome P-450-dependent mixed-function oxidase activity115 has also been identified in Clara cells. Mixed-function oxidases are involved in metabolising many environmental chemicals, including carcinogens that require catalytic activation. Intracellular levels of glutathione are important in protecting the Clara cells from injury by reactive intermediates produced by the metabolism of xenobiotics such as 3-methylindole, trichloroethylene and naphthylamine.41,116,117 Clara cell secretion of endothelin, a powerful bronchoconstrictor and vasoconstrictor, has also been demonstrated.118 The Clara cell also has stem-cell potentiality and in response to irritation gives rise to both ciliated and mucous cells.39,41,44
Brush cells
Brush cells occur infrequently at all levels of the airways from the trachea to the alveoli, where they have been called type III pneumocytes.119,120 They have a brush border of closely packed stubby microvilli up to 2 µm in length, the axial filaments of which continue into the cell without ending in a terminal web. A resemblance to intestinal cells has led to a belief that they are concerned in fluid absorption but their function remains obscure.121
Microscopy of the alveolar tissue
Beyond the terminal bronchioles, which constitute the last of the purely conductive airways, alveoli are found in progressively increasing numbers through three orders of respiratory bronchioles and two to nine orders of alveolar ducts (Figs 1.23 and 1.24).122 The walls of the alveolar ducts consist only of a thin spiral band of collagen and elastin, between which are the mouths of the alveoli, also arranged in a spiral fashion.20 The arrangement is like the coils of a spring, lengthening in inspiration and closing up in expiration. The alveolar openings usually have four straight sides whilst the alveolar walls consist of flat pentagonal or hexagonal plates arranged as a polyhedron. The alveoli are thus like boxes with one open side123: if the alveoli were spherical, their walls would stretch on inspiration and the alveolar capillaries would close. The alveolar duct system and the polyhedral shape of the alveoli act together like a concertina and thereby permit changes in volume without alterations in surface area.
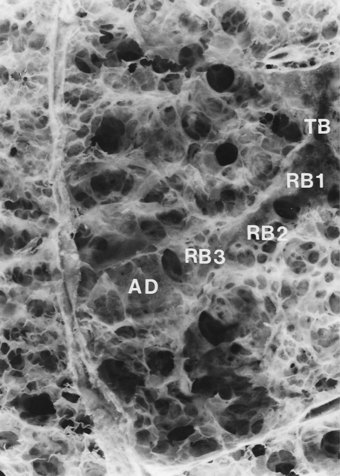
(Reproduced from Heard and Izukawa (1964)122 with permission of the late Professor B E Heard and the editor of the Journal of Pathology.)
The human adult lungs contain about 300–500 million alveoli,5,124 each measuring about 250 µm in diameter when expanded, although gravitational forces result in them being bigger in the upper than the lower parts of the lung.125 The size of the alveoli and the surface tension of their lining layer are the major determinants of distensibility and hence elastic recoil of the lungs; tissue components such as elastin and collagen contribute to a lesser degree.126 The Laplace equation indicates that the collapsing force acting on the lungs is proportional to alveolar surface tension and inversely proportional to alveolar size. Surface tension is reduced, and expansion of the lung thereby facilitated, by certain lipids, collectively known as pulmonary surfactant, that are secreted by alveolar lining cells (see below).
Small holes, the pores of Kohn, are found in the alveolar walls of many species, including humans (Fig. 1.25). Their diameter ranges from 2 to 13 µm and there are from one to seven in each alveolus. The pores are absent at birth and only develop after the first year of life. For this reason some argue that they are abnormal and represent the beginnings of emphysema. Others believe that they are normal and provide collateral ventilation, although perfusion fixation, which preserves the alveolar lining layer, demonstrates that the pores are normally plugged by this layer.127 In pneumonia, however, threads of fibrin can sometimes be seen passing through the pores from one alveolus to another. Transmission electron microscopy shows the edge of the pores to be lined by an intact epithelium.
Under the light microscope the alveolar wall is evidently rich in blood capillaries but it is not generally clear whether cells bordering the capillaries are endothelial, epithelial or interstitial. Cytokeratin immunocytochemistry shows that epithelial cells are plentiful but by light microscopy the alveolar epithelium appears to be incomplete (Fig. 1.26). It was not until 1953 that electron microscopy clarified an age-old dispute concerning the nature of the alveolar lining layer, showing that a complete simple epithelium extends throughout all alveoli (Figs 1.27 and 1.28).128 It is continuous with that of the airways. The lining epithelium is separated from the underlying connective tissue by a supportive basement membrane.
On one side of the alveolar wall the capillary is closely applied to the alveolar epithelium. Here the endothelial and epithelial basement membrane fuse and the air–blood barrier is at its thinnest, about 0.15 µm. On the opposite side of the alveolar wall, interstitial tissue separates endothelium from epithelium; this is known as the thick side of the air–blood barrier (see Figs 1.27 and 1.28). The alveolar epithelium, interstitium and capillary endothelium constitute about 30%, 40% and 30% respectively of the air–blood barrier.129
The alveolar epithelium consists of two principal cell types, variously known as I and II, A and B, small and large, non-vacuolated and vacuolated, squamous and granular respectively, which will now be considered.
Type I alveolar epithelial cells
These cells have few cytoplasmic organelles but are remarkable for their attenuated cytoplasm, which extends long distances from the nuclear zone of the cell (see Figs 1.27 and 1.28) and may even penetrate the alveolar wall so that one cell contributes to the lining of more than one alveolus.130 They each cover up to 5000 µm2 of the alveolar surface yet generally measure no more than 0.2 µm in thickness.131 Their function is to provide a complete but thin covering, preventing fluid loss but facilitating rapid gas exchange. Type I cells are connected to each other and to type II cells by tight junctions, and the alveolar epithelium provides the major barrier to fluid movement into and out of the alveolus. Nevertheless, a variety of proteins known as aquaporins that facilitate water transport across epithelia have been identified in the cell membrane of type I alveolar cells.132 Following large-volume alveolar lavage for lipoproteinosis (see p. 318) it has been found that about 53% of the residual fluid is absorbed within 1 hour.133 Numerous small pinocytotic vesicles are seen within the type I cells and macromolecules are absorbed from the alveolus by this route.134 Very fine particles reach the interstitium from the alveolar lumen by the same vesicular transport mechanism.135,136 Such absorption is probably important in sensitising the host to inhaled antigens but as a clearance pathway the alveolar epithelium is of trifling importance compared with the alveolar macrophages.
The long thin cytoplasmic processes of the type I epithelial cells are extremely sensitive to damage by various injurious agents. Together with the capillary endothelium these cells represent the component of the lung most vulnerable to damage (see Chapter 4).
Type II alveolar epithelial cells
These cells are taller and twice as numerous as the type I cells but they cover only 7% of the alveolar surface.131 They usually occupy the corners of alveoli and often all but the apex of the cell is covered by neighbouring type I cells. The free surface has blunt microvilli and the cytoplasm includes mitochondria, endoplasmic reticulum, Golgi apparatus and characteristic osmiophilic lamellar structures which represent the secretory vacuoles of pulmonary surfactant (Figs 1.29 and 1.30). These appear in fetal life at the time when surfactant can first be identified and an extrauterine existence first becomes possible. Their role in surfactant secretion has been established by experimental ultrastructural autoradiography, tracing the incorporation of surfactant precursors in a sequential fashion through secretory organelles and into the lamellar structures.137–140 Cell separation techniques enabling pure type II cell suspensions to be studied in vitro support the role of these cells as secretors of surfactant.141 Lysosomal enzymes identified in the lamellar structures are derived from multivesicular bodies which fuse with them.142,143 One of these hydrolases, phosphatidic acid phosphatase, controls an essential step in surfactant synthesis.144 Further vesicles and multivesicular bodies located immediately beneath the cell membrane are concerned in the secretion of certain surfactant-specific proteins, which are described below.145
After injury there are found occasional alveolar epithelial cells that possess features of both type I and type II cells (see Fig. 4.10, p. 139). These are flattened squamoid cells but they have the surface microvilli and osmiophilic lamellar inclusions of the type found in type II alveolar epithelial cells. Such intermediate cell forms are explained by labelling experiments which indicate that the type II cells are the stem cells from which type I cells differentiate.146,147 The type II cell is therefore not only the source of surfactant but the progenitor cell from which the alveolar wall is relined after injury to the more delicate type I cell. With chronic damage, type II cells multiply, but do not differentiate and the alveolar wall becomes lined by a cuboidal epithelium recognisable with the light microscope (see Fig. 4.8, p. 139). Animal studies have shown that in the normal lung the turnover time of type II cells is 25 days and transformation of type II to type I cells takes 2 days.147 In the developing lung a similar mechanism operates: undifferentiated cells rich in glycogen first differentiate into type II cells and these then transform into type I cells.148
Pulmonary surfactant
The tendency of the lung to contract is due partly to its elastic framework but largely to the surface tension at the alveolar air–liquid interface. Pattle reasoned from Laplace’s law that if the alveolar lining film had the surface tension of serum, the small radius of the alveolus would mean that the power required to expand the newborn’s lungs would far exceed the force of even an adult’s respiratory muscles, and concluded that the surface tension in the alveoli must be considerably less than that of an aqueous film. He went on to squeeze the fluid from mature and immature animal lungs and noted that, whereas the bubbles in the foam derived from mature animals were stable, those from fetal lungs lacked stability. This led to a series of experiments, from which it is now known that an extracellular layer that has the surface tension-reducing properties necessary to permit expansion of the lung covers the alveolar epithelium and lines the alveolus.149–152 In immersion fixed tissue, surfactant is represented by irregular fragments of osmiophilic material apparently floating free in the alveolar lumen but perfusion fixation shows that it is smooth and continuous.153 The lining is relatively thick in the corners of the alveoli and thin over the lateral extensions of the type I cells. It is biphasic and consists of an aqueous hypophase and a thin surface layer of osmiophilic lattice-work material derived from the lamellar inclusions of type II cells via intermediate tubular myelin (Fig. 1.31).
The biophysical properties of the lining layer are largely due to a series of phospholipids that are notable for their high content of saturated fatty acids, in particular palmitic acid (Table 1.3). Dipalmitoyl-phosphatidylcholine is the surfactant component which is predominantly responsible for the reduction of alveolar surface tension. It includes a small fraction of surfactant-specific proteins, upon which the surface activity is also dependent154; some of them also promote phagocytosis and are thus important in lung defence (Table 1.4).155,156 These proteins (surfactant apoproteins A–D)64 also play a role in some congenital disorders through gene mutations and subsequent deficiencies. The lining layer also contains antioxidants that are probably important in protecting the lung against inhaled pollutants.157
Table 1.3 Surfactant composition
Saturated phosphatidylcholine | 50% |
Unsaturated phosphatidylcholine | 17% |
Phosphatidylglycerol | 7% |
Phosphatidylethanolamine | 4% |
Phosphatidylinositol | 2% |
Sphingomyelin | 2% |
Other phospholipids | 3% |
Other lipids | 5% |
Serum proteins | 8% |
Surfactant-specific proteins | 2% |
< div class='tao-gold-member'>
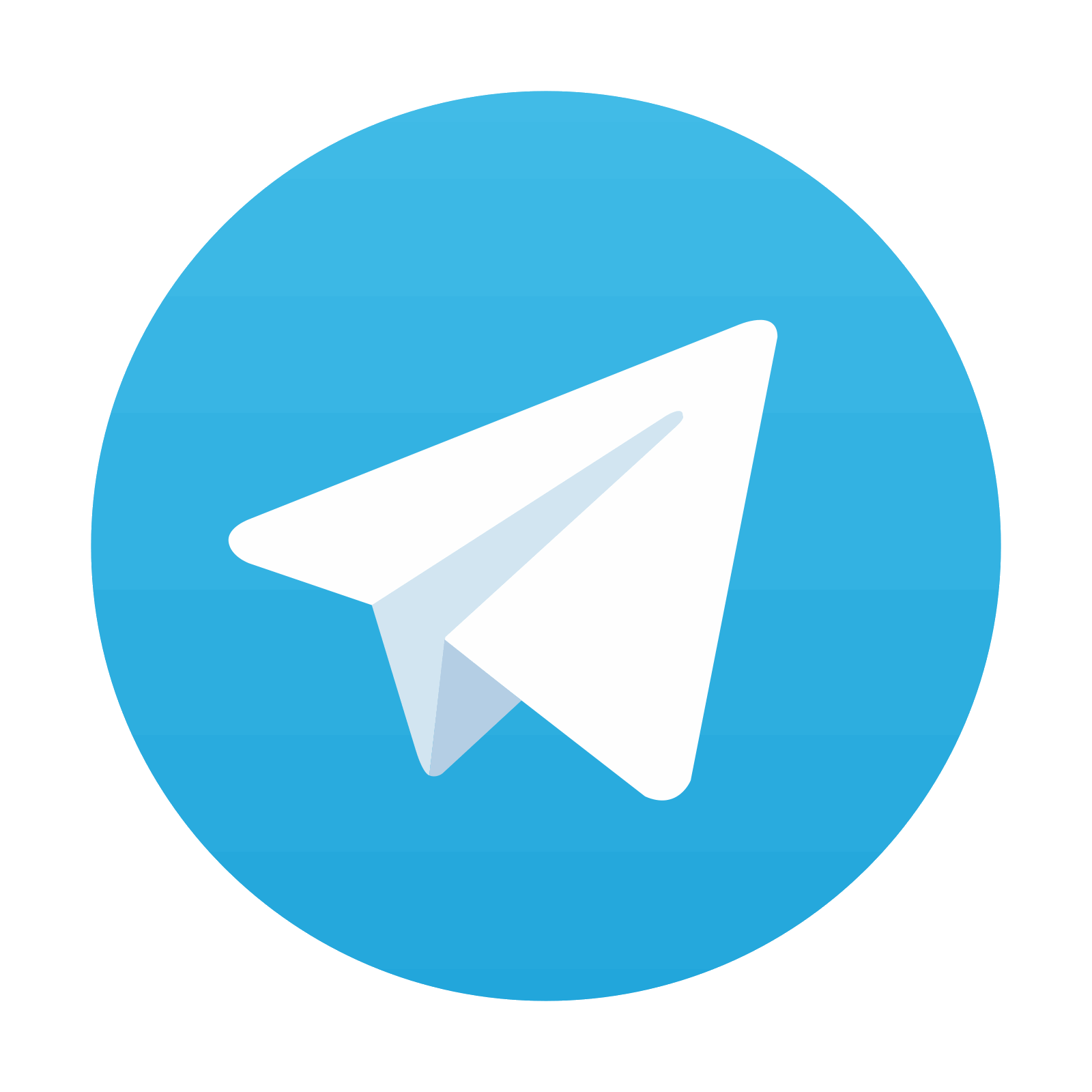
Stay updated, free articles. Join our Telegram channel
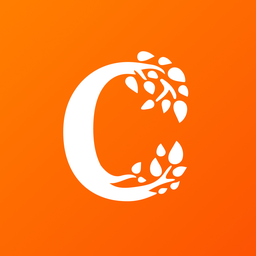
Full access? Get Clinical Tree
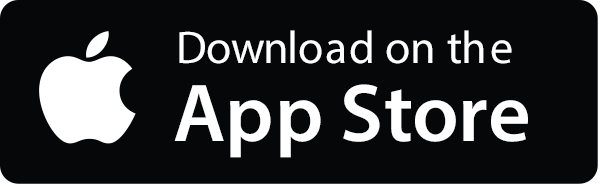
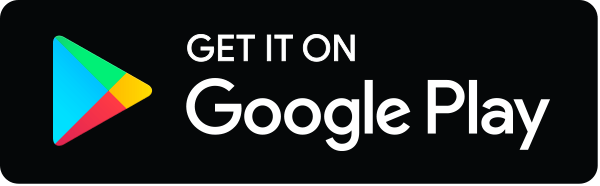