On average, human skin contains 40–70 hair follicles and 200–250 sweat ducts per square centimetre of skin. The skin appendages occupy approximately 0.1 % of the total skin surface, although this varies from region to region with, for example, the axillary, anogenital area and forehead having a larger than average concentration of hair follicles (Bronaugh and Maibach 1999). Hairs are formed from compacted plates of keratinocytes and reside in the hair follicles, which are invaginations in the epidermis. Sebaceous glands are associated with the hair follicles—usually formed as outgrowths of the follicle. They secrete an oily material, sebum, onto the skin surface. Sebum is a lipid-rich mixture which acts as a plasticiser for the stratum corneum and helps to maintain an acidic mantle of approximately pH 5 (Bronaugh and Maibach 1999). Eccrine glands are principally concerned with temperature control and are responsible for the secretion of sweat when stimulated by an increase in the external temperature or emotional factors. These glands commonly occupy approximately 10−4 % of the total skin area, and their structures ensure that they extend well into the dermis. Eccrine glands are found throughout the body, while apocrine glands are located in specific regions, including the axillae and anogenital regions.
The Subcutaneous Sensory Mechanism
The large size of the skin means that it acts as a major sensory organ for the body, particularly as it interfaces with the external environment. It provides information about the environment directly and indirectly, such as the effect of radiation on skin temperature. Fibres within the dermis form a plexus which lies parallel to the skin surface. The nerve plexus is comprised of unmyelinated and myelinated fibres. From the nerve plexus, individual fibres extend to supply particular locations in terminal branches which interconnect with and superimpose themselves upon each other in such a manner that every area in the skin is supplied by several different fibres, each of which ends in at least one particular receptor (Weddell 1941). Most of these receptors can be excited by different stimuli, but the different thresholds of stimuli required to provoke a particular receptor yield its specificity (Barlow and Mallon 1982).
The three main categories of cutaneous receptor, which are distinguished by their different sensitivities to stimuli, are the mechanoreceptors, thermoreceptors and nociceptors.
Mechanoreceptors are highly sensitive to pressure on the skin, or to movement of the hairs. Mechanoreceptors are usually described as rapidly adapting (RA) or slowly adapting (SA) types. RA mechanoreceptors include Pacinian corpuscles, which are found in both hairy and glabrous skin, and Meissner’s corpuscles, which are located in the glabrous skin of primates. Pacinian corpuscles are small oval structures found in the deeper layers of the skin. They are 0.5–2 mm long and are composed of an “onion-like” lamellar structure which is formed from non-nervous tissue. Pacinian corpuscles are able to detect mechanical vibrations at high frequencies, which may be relayed at greater than one hundred hertz per second (Brodal 1981; Sinclair 1981). The Meissner corpuscle is an encapsulated myelinated receptor found in the dermis of human glabrous skin. It is surrounded by connective tissue which is attached to the basal projections of the epidermal cells by elastin fibrils. The Meissner corpuscle allows discrimination between highly localised sensations of touch, especially in the palmar regions where they are found in their highest density (Montagna 1964). Hair follicle receptors are myelinated fibres which are primarily associated with the tactile sensations (Elliott 1969). SA mechanoreceptors, including the Ruffini endings and the C-mechanoreceptors, respond during skin displacement, including the maintenance of a discharge of impulses when the skin is held in a new position (Barlow and Mallow 1982). The Ruffini endings are encapsulated receptors found in the dermis of hairy and glabrous skin. They provide a continuous indication of the intensity of the steady pressure or tension within the skin (Brodal 1981). C-mechanoreceptors are usually found in hairy skin and have small receptive fields (approximately 6 mm2). They emit a SA discharge when the skin is indented or when hairs are moved. However, repetitive stimulation produces a rapid fall in excitability and the receptors will fail to respond after 20–30 seconds as the receptor terminals become unexcitable after this time (Barlow and Mallon 1982).
Thermoreceptors are characterised by a continuous discharge of impulses at a given constant skin temperature which increases or decreases when temperature is raised or lowered. Thermoreceptors have small receptive fields (approximately 1 mm2) and are classed as either “cold” or “warm” receptors, with the former located more towards the outer surface of the skin than the latter, at average depths of 0.15 and 0.6 mm below the skin surface, respectively (Barlow and Mallon 1982). While thermo- and mechanoreceptors contribute to the sensory quality of perceived pain, the nociceptors detect and signal high intensities of stimulation. Nociceptors generally reside at the dermo-epidermal junction and are either mechanical nociceptors (which respond to, for example, pinpricks or needles, or squeezing and crushing of the skin) or thermal, or mechanothermal, nociceptors (which respond to severe mechanical stimuli and to a wide range of skin temperatures) (Brodal 1981; Montagna 1964).
The Epidermis
The epidermis is the outermost layer of the skin. It is also the thinnest layer of the skin. Its thickness varies significantly around the body with, for example, the thickest skin being found on the weight-bearing planter surfaces (feet and hands, approximately 0.8 mm thick) and the thinnest skin being normally found on the eyelids and scrotum (0.06 mm) (Williams 2003). Despite the extensive vasculature present in deeper tissues such as the dermis, the epidermis has no blood supply and passage of materials into or out of it is usually by a process of diffusion across the dermo-epidermal junction and into the dermis. It is essentially a stratified epithelium, consisting of four, or often five, distinct layers (Fig. 1.2).


Fig. 1.2
Schematic representation of the epidermis (source BASF Personal Care and Nutrition GmbH; available at http://www.skin-care-forum.basf.com)
The Stratum Germinativum
The stratum germinativum, or basal layer, is the deepest layer of the epidermis. This metabolically active layer contains cells similar to those found in other tissues in the body and contains organelles such as mitochondria and ribosomes. It can be as thin as a single cell in depth and contains cuboid or columnar-to-oval-shaped cells which sit on the basal lamina. These cells are continually undergoing mitosis, as they provide replacement cells for the higher (outer) epidermis. Basal keratinocytes are connected to the dermo-epidermal membrane by hemidesmosomes and connect the basal cells to the basement membrane. The basal layer is also the location of other cells, including melanocytes, Langerhans cells and Merkel cells. The basal cells become flatter and more granular as they move up through the epidermis.
The Stratum Spinosum
The stratum spinosum, or prickle cell layer, sits immediately above the stratum germinitivum. It is often described with the basal layer (where the basal layer is very thin) as a single layer—the Malpighian layer. Normally, however, it is several layers thick (usually 2–6 layers) and consists of morphologically irregular cells which may range from columnar to polyhedral in structure; such a progression in morphology is common as this layer progresses upwards. Each cell in this layer possesses tonofilamental desmosomes, often called “prickles” or “spines”, which give this layer its characteristic name and extend from the surface of the cell in all directions, helping to maintain a distance of approximately 20 nm between cells. The prickles of adjacent cells link via intercellular bridges and give three-dimensional structural rigidity and increase the resistance of the skin to abrasion throughout this layer. The prickle cell layer is metabolically active despite lacking in mitosis.
The Stratum Granulosum
The stratum granulosum, or granular layer, lies immediately above the stratum spinosum and is usually one to three cells deep. It consists of flattened, granular cells whose cytoplasm contains characteristic granules of keratohyalin, which is responsible for their characteristic “granular” appearance. In the stratum granulosum, degradation of cell components becomes significant; visually, this is seen in the flattening of cells compared to the layers immediately below the granular layer, and also in a substantial decrease in metabolic activity which eventually ceases towards the top of this layer due to the degeneration of cell nuclei, which leaves them unable to carry out important metabolic reactions.
The Stratum Lucidum
The stratum lucidum sits immediately above the stratum granulosum. It is easily observed on thick skin, but may be missing from thinner skin, which is why the epidermis is often described as having either four or five layers. The stratum lucidum is often considered to be functionally indistinct from the stratum corneum and that it may be an artefact of tissue preparation and cell differentiation, rather than a morphologically distinct layer. The cells of the stratum lucidum are elongated, translucent, and mostly lack either nuclei or cytoplasmic organelles. This layer is significantly more keratinised, and contains significantly flatter cells, than the underlying layers of the epidermis.
The Stratum Corneum
The outermost layer of the skin is the stratum corneum, or horny layer. It is the final result of cell differentiation and compaction prior to desquamation and removal from the body. It is a compacted, keratinised multilayer which is dehydrated in comparison with the adjacent layers of the skin. It is, on average, 15–20 cells thick—around 10 μm in thickness when dry, although it can swell to many times its thickness when wet. The formation of keratin and the resultant cell death are part of the process of keratinisation or cornification that produces what is, in effect, the stratum corneum, the outer envelope of the body. In areas of the skin where the stratum lucidum is clearly present, the stratum corneum is usually much thicker, and this also mirrors the thickness of the viable epidermis around the body. Thus, the epidermis in those regions, such as the palms and soles, can be up to 800 µm in thickness, compared to 75–150 µm in other areas. Cells of the stratum corneum are physiologically inactive, continually undergoing a process of shedding while themselves being constantly replenished from the upward migration of cells from the underlying epidermal layers (Woolfson and McCafferty 1993a, b).
The stratum corneum is the major rate-limiting membrane of the skin and is responsible for the regulation of water loss from the body as well as limiting the ingress of harmful materials from the external environment (Scheuplein and Blank 1971). It is currently believed to consist of two alternating amorphous lipophilic and hydrophilic layers and is comparatively more lipophilic than the other epidermal layers. While generally having lower water content than other layers of the skin, the stratum corneum water content is highly variable and depends on both moisture content of the external environment of the body and the location on the body. The exceedingly dense stratum corneum may also swell to many times its own thickness in the presence of water. The water content of the stratum corneum generally decreases as the external interface is approached. The stratum corneum possesses approximately 40 % water by weight (in a relative humidity of 33–50 %). By weight, the stratum corneum is composed of approximately 40 % protein (mostly keratin) and 15–20 % lipid (triglycerides, cholesterol, fatty acids and phospholipids) although it should be noted that the exact composition will vary around the body. The stratum corneum lipids originate from a number of sources, including the discharged lamellae of membrane-coated granules, intercellular cement and the keratinocyte cell envelope (Anderson and Cassidy 1973).
The cells of the stratum corneum are flattened and elongated and are approximately 1 µm in thickness. They occupy an area of 700–1200 μm2; thus, there are approximately 105 cells per cm2. They form a closely packed array of interdigitated cells (which facilitates the formation of cohesive laminae) which are the cells stacked in vertical columns (MacKensie and Linder 1973). Each cell is contained within a mainly proteinaceous envelope rather than the conventional lipid bilayer cell membrane. This envelope provides the stratum corneum with the majority of its mechanical strength, in particular through the disulphide bonds of the intracellular keratin and by linking cells that are embedded in an intercellular lipid matrix (Matolsty 1976).
The upward movement of cellular material through the epidermis ends in the stratum corneum, which constantly sheds its outermost layers in a process called desquamation. This process involves the cleavage of intercellular bridges and may suggest a certain amount of metabolic activity and regulatory control in a layer often considered to be, to all intents and purposes, inert (Michaelis et al. 1975). Typically, the daily desquamatory loss from the stratum corneum is typically not more than 1 g, although as the rate of stratum corneum shedding is, in healthy skin, equal to the rate of epidermal cell regeneration, the stratum corneum remains approximately the same thickness.
The Stratum Corneum Barrier
The stratum corneum skin barrier has traditionally been described as a “bricks and mortar” structure (Michaelis et al. 1975; Elias et al. 1981). The “bricks” represent the tightly packed corneocytes, and they are embedded in a “mortar” of lipid bilayers. These flattened, highly proteinaceous cells are the final point of keratinocyte differentiation and are interconnected by structures called corneodesmosomes (Fig. 1.3). The “bricks” are enclosed within a continuous and highly ordered lamellar lipid bilayer. Structurally, ceramides are the most important components of this lamellar phase; they are polar lipids which contain hydroxylated alkyl side chains that, under normal conditions, are packed both hexagonally and orthorhombically. As discussed above, the keratinocyte “bricks” of the skin barrier may hydrate extensively, resulting in significant changes to the packing, structure and permeability of the stratum corneum (Michel et al. 1988; Norlen 2006, 2007; Rawlings 2003, 2010). The stratum corneum corneocytes change in their morphological and biochemical functions as they progress from the lower to higher levels of the stratum corneum. Such transitions are associated with increases in transglutaminase-mediated protein crosslinking and increased levels of intercorneocyte ceramides and fatty acids, resulting in a progression from fragile to rigid structures, described by Rawlings (2010) as the transition from “stratum compactum” to “stratum disjunctum”. This transition occurs alongside an increase in the occurrence of the protein (pro)filaggrin, which is thought to play a key role in the aggregation of keratin filaments within corneocytes (Rawlings 2010).
Despite the fundamental correctness of the “bricks and mortar” model of this stratum corneum, advances have been made in recent years, which have elaborated our understanding of the stratum corneum structure and its barrier function. New species of ceramides and the synthetic pathways that generate them are still being identified (Rawlings 2010). Cryoelectron microscopy studies have proposed the existence of a single gel phase model for the stratum corneum lipids while failing to show the expected presence of the trilamellar-conformation long periodicity phase (Norlen 2007). Bouwstra et al. (1998) suggested that the stratum corneum lipid phase could be described by a “sandwich model” which explains differences observed in stratum corneum lipid packing, particularly with regard to differing periodicity phases. This model highlights the importance of a fluid phase within the stratum corneum which may be dictated by the presence of ω-esterified long-chain acylceramides.
Ultimately, the stratum corneum cannot be considered as a homogenous tissue as it exhibits clear changes as it progresses outwards from the body—the transition from “compactum” to “disjunctum”. This transition may be exemplified by a transition in the packing of ceramide side chains from a more tightly packed orthorhombic phase to a less tightly packed hexagonal phase which becomes increasingly present closer to the skin surface. In addition, at the skin surface the lamellar phase is normally missing as it becomes increasingly amorphous in nature at this point (Pilgram et al. 1999, 2001; Rawlings 2010).
Routes of Permeation of Exogenous Chemicals Across the Stratum Corneum
It is widely understood that the main route for exogenous chemicals to pass into and across the stratum corneum is via the lipid pathway. Despite being a longer and more torturous route across this layer, it does not require the multiple partitioning steps associated with the transcellular pathway. Rather, it simply relies on partitioning of the penetrant into the stratum corneum lipids from its formulation or vehicle (if the chemical is applied in this manner) and subsequent diffusion across the lipid bilayer towards the underlying viable epidermis. The appendageal route of absorption—permeation, for example, via the hair follicles and sweat glands—is of limited significance in the overall permeation process as these structures occupy, on average, 0.1 % of the total skin surface and therefore provide a limited target for permeation. Further, structures such as sweat glands are often morphologically similar to the remainder of the skin surface, limiting the viability of these structures as a route of absorption even more as absorption also has to compete with an opposing outward flow of liquid when active. Thus, the stratum corneum lipids play the dominant role in limiting or controlling percutaneous absorption. However, it should be noted that while it is the most important route, it is not the only route, and that other routes of administration will contribute to the overall process of skin permeation (Moss et al. 2012).
Percutaneous Permeation—Mechanisms of Absorption
For a chemical to pass into and across the skin, and become systemically available, it must undertake a series of partitioning steps. The chemical is usually presented to the skin surface in a formulation or vehicle from which it must partition onto the skin surface, where those molecules in contact with the stratum corneum will begin to partition. Depending on their properties, the nature of both the vehicle or formulation and the penetrant can play a key role in determining the rate at which the chemical penetrates the skin. For example, if the penetrant has a high affinity for the formulation, then it may remain there, whereas if it has a low affinity for the formulation (or a higher affinity for the stratum corneum), then it may partition into the skin more readily. Thus, the penetrant adjacent to the skin surface will permeate into the stratum corneum, a process which is dependent on the random movement of the penetrant from the bulk of the vehicle to the surface of the skin, which again may be influenced by the nature of the vehicle or formulation. Once the penetrant has diffused into the stratum corneum, it will begin to diffuse through this layer, with the rate of diffusion again depending on the physicochemical properties of the penetrant. This may occur via any of the three main routes described above (intracellular, intercellular and transappendageal; shown in Fig. 1.4). Permeation may be either via a specific route or a combination of any of the available routes. The next significant challenge to permeation is at the junction of the stratum corneum and the viable epidermis. At this point, the underlying tissues may be broadly differentiated from those above as they are more hydrophilic than the outer layers of the epidermis, and the stratum corneum in particular. This results in a further partitioning step and diffusion into the viable epidermis, therefore partitioning between the viable epidermis and the dermis. Finally, partitioning from the dermis to the capillary system results in the penetrant being removed to the systemic circulation.


Fig. 1.4
Pathways of drug penetration through skin
The transepidermal route, via the intact stratum corneum, is the main route through which penetrants may enter, as it provides the major area available to a potential penetrant. The stratum corneum has been morphologically and functionally represented by the “bricks and mortar” model (Elias 1988). The “bricks”, or corneocytes, of this model provide a dense, fibrous, proteinaceous network, with the “mortar” forming a predominately lipophilic matrix. Successful permeability of the intact stratum corneum has been shown to relate predominately to lipophilic materials and depends to a large extent on the oil/water partitioning property of a particular penetrant, usually measured as the octanol–water partition coefficient (P, or more commonly log P). Relationships between permeability and partition coefficients have classically been demonstrated by various investigations (Treherne 1956; Blank 1964; Scheuplein 1965, 1967; Scheuplein et al. 1969; Barry 1983; Williams 2003).
The other potential route for transdermal penetration is the transappendageal, or “shunt”, route through skin appendages including hair follicles and sweat ducts. These structures may lack a horny layer and, in theory, offer low resistance to permeation compared to other routes (Barry 1983). Scheuplein (1967) concluded that transappendageal absorption may be important in the early “lag” period of the penetration process. However, while diffusion through glands is generally considered to occur, the rate and extent of permeation is, in most cases, negligible due to the small area they occupy on the surface of the skin, and the current of secretions passing to the outer surface as mentioned earlier, which is often mediated by valve mechanisms at the openings of the glands (Barr 1962; Heuber et al. 1992, 1994). Ultimately, however, successful permeation of exogenous chemicals via the shunt route depends predominately upon the physicochemical properties of the penetrant as well as the nature of the stratum corneum and may be more successful for some penetrants than for others. In addition, other factors may influence the penetration process. For example, the potential for protein binding, which may occur in the stratum corneum, will contribute to the reservoir effect associated with that layer. Metabolic activity may see some, or potentially all, of the permeant degraded before it reaches the blood vessels. There is also potential for permeants to pass into deeper layers of the skin, including the subcutaneous fatty layer, or even into muscle tissues underlying the skin.
Theoretical Considerations
Diffusion is “a process of mass transfer of individual molecules of a substance, brought about by random molecular motion and associated with a concentration gradient” (Martin et al. 1983). Diffusion through a non-porous membrane, such as the stratum corneum, occurs when the diffusant dissolves in the bulk membrane or solvent-filled pores of the membrane. Such diffusion is influenced by the size and physicochemical properties of the penetrant and the nature of the membrane, and possibly also the formulation or vehicle particularly if it exerts a change on the nature of the membrane. While the three layers of the skin (the epidermis, the dermis and the subcutis) each have their own diffusion coefficient, diffusion through any layer other than the stratum corneum is generally considered to be negligible and, as such, they are normally treated together and represented by a single diffusion coefficient.
Thus, total diffusional resistance of the skin is generally attributed to the stratum corneum under passive diffusion, and therefore, Fick’s first law of diffusion may be applied (Martin et al. 1983; Moss et al. 2002):

where

(1.1)
J
is the rate of transfer per unit area of the surface (i.e. the flux);
C
is the concentration of the diffusing substance;
x
is the spatial coordinate measured normal to the section; and
D
is the diffusion coefficient, or diffusivity.
The dermal permeability coefficient, kp, is defined by the equations:

or

(1.2)
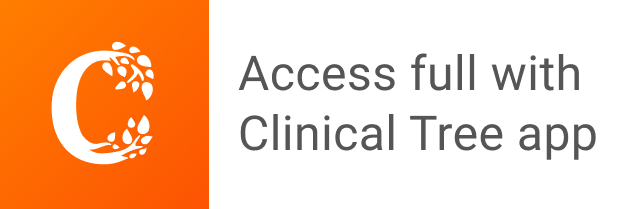