There are two main types of diffusion cells: static and flow-through cells. The vast majority of in vitro percutaneous absorption experiments are conducted using upright static glass diffusion cells, known as Franz or Franz-type cells (Franz 1975). These involve the partition of the permeant into a “static” receptor compartment of a fixed volume that is maintained at a controlled temperature and is continually stirred throughout the experiment to ensure complete mixing and to avoid the presence of any “dead” zones or diffusion gradients within the receptor compartment. Franz-type diffusion cells are found in a range of designs and sizes (Fig. 2.1) and are therefore very flexible, with particular cells being chosen for particular purposes (e.g. when a different surface area might be required, or when the receptor compartment may need to be varied based on the solubility of a particular permeant). The simple design of the upright cells allows a wide range of formulations to be applied to the skin surface, including solutions, creams, ointments and various other semi-solid materials of a pharmaceutical or cosmetic nature. A variation on the Franz-type cells is the side-by-side cell, which allows both compartments to be stirred at the same time, although the range of formulations suitable for use in such cells is clearly limited.
One of the main perceived limitations of the Franz-type cells is their susceptibility to “sink conditions”. As described above, the diffusion process requires a gradient to be established, and maintained, across the membrane that divides the donor and receptor compartments. Thus, in a static diffusion cell, it is important to maintain a concentration gradient such that, normally, the concentration of the permeant of interest is not greater than 10 % of its saturated solution in the receptor compartment (Martin et al. 1983; Anissimov and Roberts 1999; Roberts and Cross 1999; Roberts et al. 1999). Flow-through diffusion cells, often called “Bronaugh cells”, offer an alternative approach to the issue of sink conditions and attempt to mimic in vivo conditions by utilising a constantly perfusing receptor compartment, flowing usually at 1–2 ml/h, which aims to mimic the blood flow beneath the skin (Bronaugh et al. 1999a, b). This is normally achieved with the use of a peristaltic pump which is connected to tubing that supplies a compartment beneath the membrane. The receptor fluid flows into a receptacle and is analysed, either offline or, more infrequently, via an online flow-through method of analysis. Either arrangement provides the facility for substantial automation of sample collection. This arrangement ensures that sink conditions are maintained throughout the experiment and that the receptor phase does not have to be replaced should a penetrant be rapidly absorbed. While flow-through cells are more flexible (i.e. in terms of automation), they are more complex and substantially more expensive than Franz cells. Consequently, the use of Franz-type static diffusion cells is more common than the use of flow-through cells. In either case, most experiments use between 6 and 24 cells per experiment in order to ensure reproducibility.
As mentioned above, the cell design can influence the formulation type that can be applied to it. For example, the side-by-side cells, while having the advantage of even stirring and mixing in both donor and receptor compartments, are limited in the type of formulation that can be applied—pharmaceutical dosage forms such as creams, gels, ointments and patches are very difficult to apply in this manner. Thus, the cell design and the protocol used can all affect the outcome—the measurement of percutaneous absorption and the cell design should be considered when considering the uniformity of data that are to be used in the construction of mathematical models of skin absorption.
For example, the manner in which the product is applied to the skin surface is important to consider in model construction, as each individual experiment may require specific amounts, or even frequencies of application, or they may require that the donor phase is occluded, or not occluded—the experimental protocol used is dependent on the nature of the penetrant and the context of the experiment. An experiment might wish to examine the occupational hazard of a chemical being exposed to the skin—in, for example, the context of crop spraying of a pesticide. Therefore, an experimental protocol might use a non-occluded experiment (to mimic the potential of the solvent, the pesticide is delivered in evaporating vehicle) and a fixed, or finite, dose which would represent the amount commonly sprayed and therefore the amount that the skin (per unit area) might be exposed to. Equally, an experiment may wish to determine the “worst-case scenario” for the application of a new chemical to the skin, and, in such circumstances, an occluded experiment may be favoured as this generally increases the permeation of a topically applied chemical (Zhai and Maibach 2001). Such a protocol might also use an excessive amount of chemical—an infinite dose—that aims to maintain the maximum flux of this chemical across the skin, in order to determine permeation in the “worst case”. Such an arrangement also finds use when examining the fundamental behaviour of a material and is used to establish the steady-state rate of permeation across the skin. Infinite doses are also used when considering the effects of formulation ingredients that alter the barrier function of skin (penetration enhancers) on the penetration of a chemical (Williams 2003).
The application of an infinite dose to the surface of the skin means that there is little or no significant change to its concentration (in the donor phase) or thermodynamic activity throughout the experiment. However, once permeation commences, the donor phase becomes depleted and this scenario is no longer valid. In a practical sense if the concentration in the receptor does not exceed 10 % of the saturated solubility, an infinite dose can be assumed. Further, supersaturated systems have been employed (e.g. Raghavan et al. 2001; Dias et al. 2003; Raghavan et al. 2003) to resolve this issue; the donor phase is composed of a saturated solution of the penetrant of interest, into which is also added an additional amount of the penetrant, which will be present as a solid. As diffusion into and across the skin commences, the donor phase can maintain the saturated solution should the conditions, including the rate of solubility, permit it to do so (and, therefore, maintain in theory the same concentration and thermodynamic activity) throughout the duration of the experiment.
Conversely, in a finite-dose experiment, a fixed amount of a material is applied to the skin surface and it is expected that it will deplete during the course of the experiment. This may additionally be facilitated by whether or not the donor phase is covered (occluded) during the experiment, as a non-occluded system may result in the evaporation of any solvent which may alter, both positively and negatively, the permeation of the material. Such experiments are very useful in that they allow an estimation of permeation that is more realistic and which more accurately represents the “in-use” performance of a product.
Temperature
The temperature at which an in vitro percutaneous penetration experiment is conducted is normally 37 °C, maintained by the use of circulating water, as discussed above. This results in a temperature of 32 °C at the skin surface. It is important to maintain this temperature throughout the experiment as skin permeability may vary significantly with even small changes in temperature (Chilcott et al. 2005). However, data sets collated for several studies (i.e. Moss et al. 2009; Lam et al. 2010) show that, while the vast majority of experiments that constitute those data sets have been conducted at either 32 or 37 °C, a significant number of experiments were conducted at temperatures of 31–32, 25–31 and 22–30 °C. The implications of this are discussed in later chapters.
Formulation and Solubility Factors
The nature of any formulation applied to the skin must be considered when using the information from an in vitro diffusion cell experiment to construct a mathematical model of permeation. If a chemical applied to the skin, whether it is the permeant of interest or a material formulated to facilitate the delivery of the permeant, alters the permeability of the skin, then this can significantly influence permeability. For example, certain solvents will alter the permeability of the skin barrier. These include water (whose hydration effects are, comparatively, somewhat limited), ethanol, propylene glycol and other organic solvents. These materials may be used to facilitate the delivery of drugs into or across the skin. For example, urea has also been shown to increase the hydration of the stratum corneum and increase the onset of erythema (Hellgren and Larsson 1979; Beastall et al. 1988). Urea has also been employed clinically to enhance hydrocortisone penetration from commercially available products, such as Alphaderm® and Calmurid®.
However, in the context of mathematical modelling, most of the data used to construct models come from experiments where formulation, other than solvents, is not considered (this is a limitation that will be discussed in detail later). Generally, the permeability data from which models have been constructed use aqueous or water–ethanol solvents in the donor phase, and few studies have explored formulation matters in detail (Pugh et al. 2005; Ghaforuain et al. 2010a, b; Moss et al. 2011). This also applies to ionisation, which is not generally considered by most models of percutaneous absorption.
The main issue with solvent effects in percutaneous absorption experiments that are relevant to the construction of mathematical models is the use of non-aqueous materials in the receptor compartment of the diffusion apparatus. Ideally, the receptor fluid should mimic as closely as possible the nature of the sink into which the permeant will diffuse when applied topically in vivo—the viable tissues of the skin and the blood supply. Chapter 1 described the structure of the skin and how its substantial vascularised structure provides a very efficient means of rapidly removing permeants and maintaining an effective diffusion gradient across the skin. Thus, an efficient receptor fluid in an in vitro experiment should similarly aim to maintain sink conditions and while not altering the nature of the membrane. In general, the vast majority of experiments use similar receptor fluids, although significant differences do occur and should be considered, both for their impact on permeation and for their impact on the subsequent, and in most cases unconnected, development of mathematical models. Most receptor fluids are based on buffered aqueous systems (or, in the case of non-ionised species, simple aqueous systems). Such systems are usually buffered at pH 7.4, usually with a phosphate buffer, to mimic physiological pH. However, there are exceptions, such as the use of a receptor phase buffered at pH 5 which is used to measure the permeability of aluminium across human skin (Mistry et al. 2013). This study used a receptor phase buffered at pH 5 as aluminium otherwise forms an insoluble oxide, which is difficult to analyse. Antimicrobial agents are often added to receptor fluids to maintain the integrity of the receptor compartment. If the penetrant of interest is lipophilic, then organic solvents, such as ethanol or propylene glycol, can be used, often in concentrations up to 25 %, with the remainder of the fluid being composed of water or a buffered aqueous system. Other solubilising agents have been used in receptor phases, including various surfactants and protein (i.e. bovine serum albumin). Care should be taken to consider the effect that the receptor compartment, through back-diffusion into the skin, may exert on barrier integrity, hence the suggestion to measure barrier integrity at the beginning and the end of an experiment. Therefore, the selection of a receptor fluid may be experiment-specific as different experiments aim to evaluate the permeability of a range of chemicals. Thus, care should be taken when using such information to construct models.
Detection of the Permeant
Once the receptor compartment fluid has been collected, it will be analysed by a number of commonly used methods. These include “cold” chromatographic methods (predominately high-performance liquid chromatography, HPLC, which is increasingly being coupled with mass spectrometric (MS) methods of analysis) or “hot” radiolabelled methods, usually by 14C or 3H-labelling of the permeant. This latter method is expensive, requires the use of licensed premises and, in the case of 3H-labelled materials, offers the opportunity for tritium exchange between the material of interest and other materials in the analytical sample. HPLC conversely is comparatively inexpensive and widely used as a method of detection in biologically derived samples. In simpler diffusion experiments, such as those where artificial membranes (i.e. PDMS) have been used, UV spectrometry has been employed. While each method has its advantages and disadvantages, they all, if used and validated appropriately, can quantify the permeability of a material of interest such that accurate diffusion profiles can be produced, from which flux and permeability coefficients can be derived. Flux (as concentration/surface area/time, i.e. mg/cm2/h) is usually the gradient of the steady-state portion of the diffusion profile. The permeability coefficient, k p, is, in essence, the concentration-corrected flux and has units of distance and time (i.e. cm/s). Further, the lag time can be determined by extrapolating the steady-state section of the graph to the x-axis (the axis of the diffusion plot on which time is normally plotted). It is this data, mostly k p but, in some cases flux (i.e. Magnusson et al. 2004), that is used by those constructing mathematical models of percutaneous absorption.
Conclusions
There exists a wide range of potentially conflicting experimental protocols for the determination of percutaneous absorption. All these methods are entirely valid in that they have been developed with specific penetrants in mind and not with the development of mathematical models, the latter activity often being unconnected to the original experiments. Thus, their results should be considered in this context—so too should their use in the development of the mathematical models and their limitations clearly enunciated and understood. For example, as we will see in later chapters, data sets have been compiled, and mathematical models developed, from experimental data that have been collated from different sources, which often use differing experimental protocols.
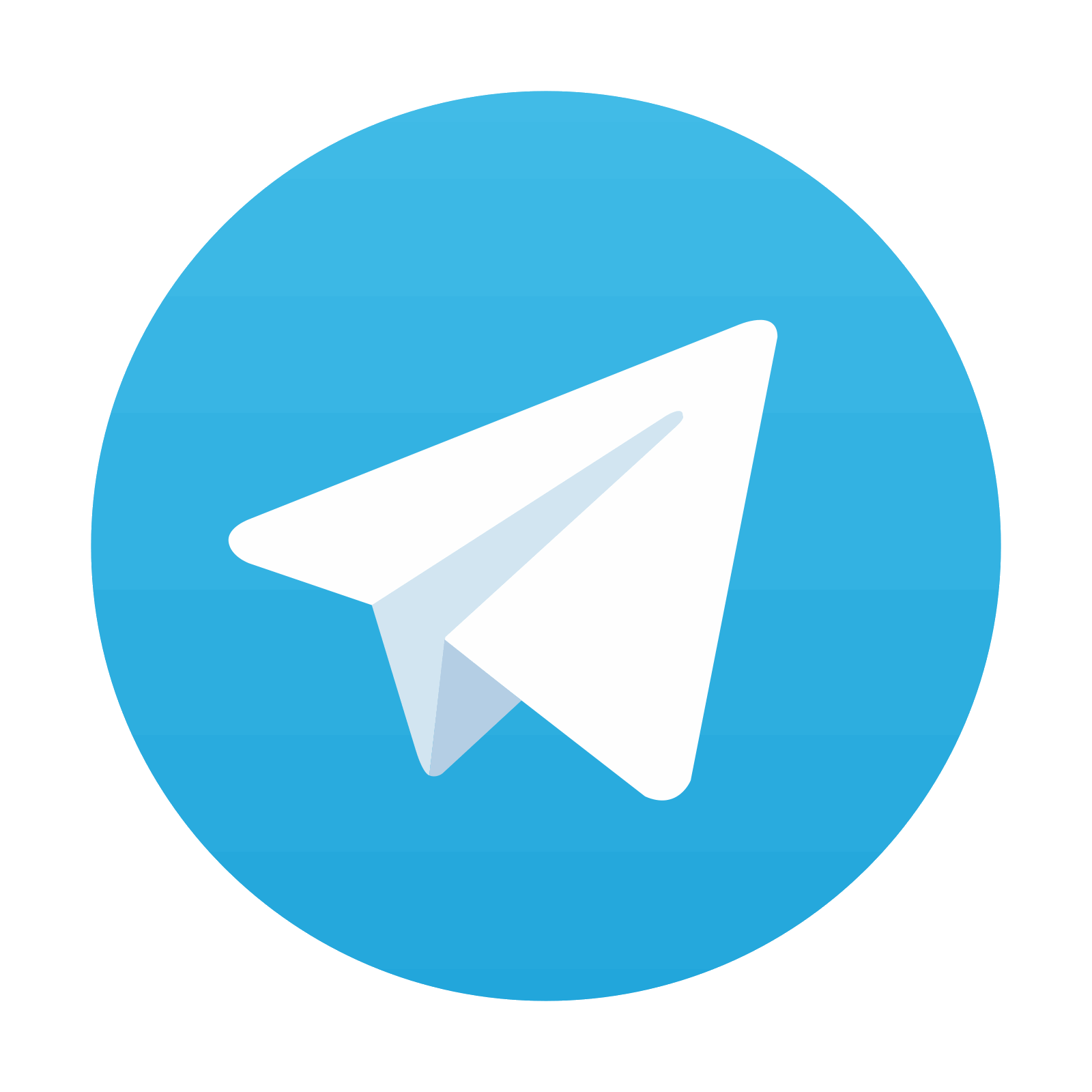
Stay updated, free articles. Join our Telegram channel
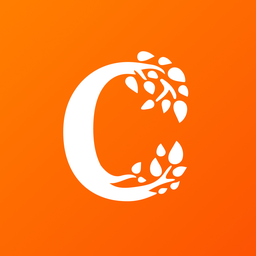
Full access? Get Clinical Tree
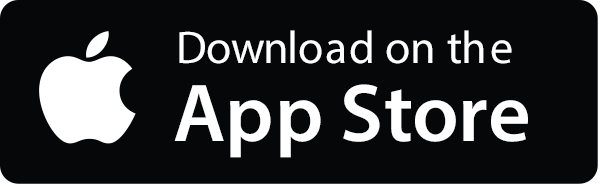
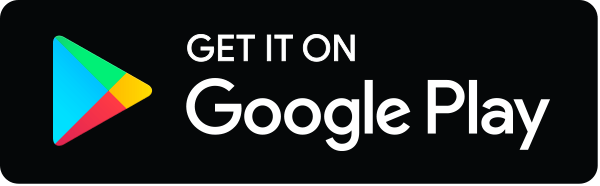