The biochemical changes associated with the hypoperfusion of shock occur even with compensation. Because of the potential difficulties in diagnosing compensated shock, an arterial and/or venous blood gas analysis including base deficit and lactate should be obtained for every patient suspected of being in shock. Additionally, any patient with a lactate of ≥4 mmol/L or base deficit of ≥6 mEq/L should be considered to be in shock until proven otherwise.17,20,21
Future Measures: Although each of these factors can be used to characterize shock, no single measurement has been determined to be optimal or when used singly to be always accurate for identification and treatment of shock. Currently, other measurements are being investigated that demonstrate promise. These markers include measurements of central and mixed venous oxygen saturations, end-diastolic cardiac volume indices, and specific noninvasive end-organ tissue oxygen saturations.22–28
TYPES OF SHOCK
Although several different classifications for shock have been described, the most widely accepted classification of shock was proposed by Weil and Shubin in 1971.6 This classification divides the shock syndrome into four distinct categories (Table 9-2). Despite this separation, however, there is considerable overlap between the categories, with some patients presenting with more than one factor at the same time. Given this overlap, it is helpful to evaluate the hemodynamic pattern in order to elucidate the etiology and manage the patient (Table 9-3).
Hypovolemic Shock
2 Hypovolemic shock is the form of shock most commonly encountered in surgical practice (Table 9-2). The essential feature is a reduction in intravascular volume to a level that prevents the heart from being able to pump sufficient blood to vital organ systems. Substantial blood (>20% circulating volume) or plasma (via soft tissue, enteric sequestration, gastrointestinal, urinary, or other insensible losses) losses are required to produce this syndrome.
The signs and symptoms of shock vary with both the severity and duration of fluid loss. A review of the Advanced Trauma Life Support classification system of the American College of Surgeons is useful to comprehend the manifestations and physiologic changes associated with hemorrhagic shock in adults.29 Blood volume is estimated at 7% of ideal body weight, or approximately 4,900 mL in a 70-kg patient (Table 9-4).
Class I: Mild hemorrhage, up to 15% of total blood volume. This condition is exemplified by voluntary blood donation. In the supine position, there are no measurable changes in heart or respiratory rates, blood pressure, or pulse pressure. Capillary refill is normal. This degree of hemorrhage requires little or no treatment, and blood volume is restored within 24 hours by transcapillary refill and the other compensatory methods.
Class II: Loss of 15% to 30% of blood volume (800 to 1,500 mL). Clinical symptoms include tachycardia and tachypnea. The systolic blood pressure may be only slightly decreased, especially in the supine position, but the pulse pressure is narrowed (because of the diastolic increase from adrenergic discharge). Urine output is reduced only slightly (20 to 30 mL/hr). Mental status changes (e.g., anxiety) are frequently present. Capillary refill is usually delayed. Patients with class II hemorrhage usually can be resuscitated with crystalloid solutions, but some may require blood transfusion.
Class III: Loss of 30% to 40% of blood volume (up to 2,000 mL). Patients with class III hemorrhage present with inadequate perfusion that is obvious; marked tachycardia and tachypnea; cool, clammy extremities with significantly delayed capillary refill; hypotension; and significant changes in mental status (e.g., confusion, combativeness). Class III hemorrhage represents the smallest volume of blood loss that consistently produces a decrease in systolic blood pressure. The resuscitation of these patients frequently requires blood transfusion in addition to administration of crystalloids.
Class IV: Loss of more than 40% of blood volume (more than 2,000 mL), representing life-threatening hemorrhage. Symptoms include marked tachycardia, a significantly depressed systolic blood pressure, and narrowed pulse pressure or unobtainable diastolic pressure. The mental status is depressed and the skin is cold and pale. Urine output is negligible. These patients require immediate transfusion for resuscitation and frequently require immediate surgical or other (e.g., angiographic embolization) intervention.
In practice, individual susceptibility to blood loss varies greatly and is affected by age, pregnancy, pre-existing disease, prescription and nonprescription medications (e.g., beta blockers), adequacy of compensatory mechanisms, and other factors that are poorly characterized. Presence of these factors should lead the caregiver to consider early use of invasive monitoring as an adjunct to appropriate fluid resuscitation.
Hypovolemia due to plasma losses may also lead to hypovolemic shock. The clinical findings of hemorrhagic shock are typically present, but significant differences do exist. Hemoconcentration, elevated blood urea nitrogen (BUN) and creatinine, and hypernatremia are typical of acute plasma and/or free water losses and are not necessarily present in other forms of shock. Appropriate evaluation of preload and urine output should be followed, with specific considerations for unique electrolyte abnormalities associated with specific plasma and fluid losses (e.g., gastric vs. colonic losses).
Cardiogenic Shock
3 The clinical definition of cardiogenic shock is decreased cardiac output with tissue hypoperfusion, despite presence of adequate intravascular volume. It is caused by a primary problem with the cardiac muscle, electrical conduction system, or valves. The most common cause is anterior wall myocardial infarction, although in surgical patients it is often precipitated by pulmonary embolus, myocardial contusion, or pulmonary hypertension.
Distinguishing cardiogenic shock from other shock etiologies is occasionally difficult. It is not uncommon to see combined cardiogenic and traumatic shock in the elderly patient, with one often being the precipitating event for the other. The hallmarks of the hemodynamic and neuroendocrine response to systemic hypoperfusion from other causes are also typical of cardiogenic shock. Eliciting a history of pre-existing cardiac disease, and physical findings such as pulmonary rales, cardiac murmurs, an S3 gallop, and jugular venous distention may be helpful. An electrocardiogram may detect significant ischemia or other pathology. A chest radiograph may reveal bilateral pulmonary infiltrates typical of cardiogenic edema, and cardio-specific serum tests (troponin I and creatine phosphokinase [CPK]) may indicate myocardial damage.
Manifestations of cardiogenic shock develop as a consequence of failure of peripheral perfusion, the associated adrenergic response, and the inability of the heart to accommodate blood returning from the lungs and the periphery. In the absence of sepsis or tissue injury, however, there is not usually an associated increase in the metabolic needs of the peripheral tissues. Sympathetic-mediated constriction of the peripheral vasculature attempts to maintain central blood pressure and perfusion of cerebral and coronary circulations. The clinical findings of cardiogenic shock may thus be similar to those of hypovolemic shock because both involve induction of the adrenosympathetic response.
Table 9-2 Classification System and Causes of Shock
Diminished or ineffective contractile activity of the right or left side of the heart allows blood to accumulate in the respective venous circulations. Shock from an acute left ventricular myocardial infarct occurs when more than 40% of the left ventricle is involved and may be present in approximately 20% of Q-wave infarcts.30 Shock from an acute right ventricular myocardial infarct, on the other hand, is rare and only occurs in approximately 10% of all inferior wall infarcts.31 Not only does the diagnosis of each infarct vary, but also the treatment and support vary significantly.
Table 9-3 Hemodynamic Patterns in Shock
In any patient in shock, especially in those with compromised cardiac function, consideration should be given to the institution of mechanical ventilation. The work of breathing can be considerable, especially for the patient in a state of agitation or distress. Oxygen needs are decreased through intubation and mechanical ventilation. In this manner, the patient can be comfortably sedated with a secure airway; the work of breathing is undertaken by the ventilator, and gas exchange can be optimized. If there is a tenuous balance between myocardial oxygen needs and availability, the balance can thus be shifted in the patient’s favor.
Like other forms of shock, cardiogenic shock tends to be self-perpetuating. Myocardial perfusion depends on the pressure gradient between the coronary artery and the left ventricle and the duration of diastole. Both are compromised by the hypotension and tachycardia that characterizes this condition. High-volume fluid resuscitation, sometimes necessary for treatment of other forms of shock, is poorly tolerated and likely to be detrimental to an individual with the compromised myocardial function of cardiogenic shock.
Extracardiac Obstructive Shock
4 A subset of patients with cardiogenic shock do not have intrinsic cardiac disease but have pump failure due to extrinsic compression that limits diastolic filling and cardiac output. Cardiac tamponade, tension pneumothorax, diaphragmatic herniation of abdominal viscera, mediastinal hematoma, and, occasionally, positive-pressure mechanical ventilation may all precipitate cardiogenic shock by exerting constricting pressure on the myocardium. The key to diagnosis and appropriate therapy is the physical examination, supplemented by use of appropriate diagnostic modalities.
The classic physical findings of cardiac tamponade, Beck triad, are hypotension, muffled heart sounds, and jugular venous distention. Unfortunately, these nonspecific clinical findings are seldom present together. Elevated jugular venous pressure, noted by either physical examination or measurement of CVP, although not specific for cardiac compression, is usually present. Pulsus paradoxus can be useful for the diagnosis of cardiac tamponade. Although it may be caused by other conditions, it is virtually always present in patients with tamponade.
Given the difficulty in evaluation of these patients, especially in combination with other shock states, early diagnostic evaluation, both invasive and noninvasive, of cardiac function is warranted. Recent guidelines suggest that any patient with concern for primary or secondary cardiac dysfunction should undergo early ultrasound evaluation. This early evaluation not only evaluates the primary cardiac function but also can lead to the early diagnosis and management of secondary causes of cardiac dysfunction including cardiac tamponade and pulmonary embolism.
Table 9-4 Classification of Hemorrhagic Shock
Table 9-5 Systemic Inflammatory Response Syndrome
Distributive Shock
Distributive shock occurs in a state of inappropriate oxygen utilization associated with the systemic inflammatory response syndrome (SIRS). Classically, SIRS is triggered by sepsis, but SIRS is associated with other immune processes including trauma, pancreatitis, and other types of tissue injuries. However, other types of distributive disturbances can occur unrelated to inflammation that may be directly due to loss of vascular tone from spinal cord injury, endocrine dysfunction, or anaphylaxis.
Septic Shock
5 Septic shock is defined as a SIRS response to infection in conjunction with arterial hypotension, despite adequate fluid resuscitation.32 It occurs when bacterial products interact with cells of the immune system, leading to elaboration of mediators that cause circulatory disturbances and direct and indirect cell damage leading to the clinical manifestations of SIRS (Table 9-5).33 Hemodynamic changes are defined as early (warm or hyperdynamic) or late (cold or hypodynamic). These stages are primarily characterized by the degree of ventricular contractility and peripheral vasomotor impairment present, but can be misclassified if not appropriately evaluated. Early septic shock is distinguished by peripheral vasodilation, flushed and warm extremities, and a compensatory elevation in cardiac output. Although an increase in venous capacitance diminishes venous return to the heart, cardiac output is maintained via tachycardia and the decrease in afterload due to systemic vasodilation.
Late septic shock is characterized by impaired myocardial contractility due to local and systemic release of cardiac depressants, worsening peripheral perfusion, vasoconstriction, extremity mottling, oliguria, and hypotension. Peripheral oxygen utilization may be severely impaired by bacterial toxins, such as lipopolysaccharide (LPS) and the inflammatory products of the host’s own immune response, resulting in metabolic dysfunction and acidosis despite a high systemic oxygen delivery. This inappropriate oxygen utilization and systemic shunting lead not only to confusion regarding the adequacy of resuscitation but also to progressive cell death. Together, both systemic hypoperfusion and the altered tissue metabolism create a vicious cycle that propagates the inflammatory response initiated in reaction to the initial infectious challenge leading to progressive cellular injury.
Due to both volume deficits and cardiovascular dysfunction, persistent perfusion deficits are common and contribute significantly to multiple organ failure and mortality. In fact, the fluid volume required for treatment may exceed that required for treatment of other forms of shock due to persistent microvascular endothelial capillary leak. As a result of this profound leak, interstitial and total-body fluid balances become extreme, leading to the potential development of marked hypoxia and the abdominal compartment syndrome (ACS).
Although appropriate early resuscitation and cardiovascular support are essential to the treatment of septic shock, as important are early infection source control and appropriate administration of antimicrobials. In fact, numerous investigators have demonstrated that even a few hours delay in initiation of antimicrobial therapy is associated with a significant increase in mortality.34
Traumatic Shock
The major contributor to shock after injury is hypovolemia due to hemorrhage. Even when hemorrhage ceases or is controlled, patients can continue to suffer loss of plasma volume into the interstitium of injured tissues and develop progressive hypovolemic shock. In addition, tissue injury evokes a broader pathophysiologic immunoinflammatory response and a potentially more devastating degree of shock than that produced by hypovolemia alone.
The degree to which direct tissue injury and an inflammatory response participate in the development and progression of traumatic shock distinguishes it from purely hypovolemic shock. Thus, traumatic shock results from direct tissue or bony injury, resulting in not only hypovolemia caused by fluid and blood loss but also an immunologic and neuroendocrine response to tissue destruction and devitalization. This combined insult complicates what might otherwise be straightforward hemorrhagic shock by inducing a systemic response that utilizes many of the inflammatory mediators present in septic shock.35 These mediators propagate and intensify the effects of the initial hypovolemia and make subsequent multiple organ failure far more likely than occurs with hypovolemic shock alone.
Although this condition can lead to increased fluid requirements, common problems associated with this condition such as rhabdomyolysis should be aggressively evaluated and treated with optimal resuscitation.36 In addition, common patient characteristics are known to alter traumatic shock resuscitation, in particular, morbid obesity that can result in delayed correction of metabolic acidosis and increased risk for organ dysfunction.37
Thus, initial management of the seriously injured requires the assurance of an airway, breathing, and circulation; later management requires appropriate volume resuscitation and control of ongoing losses. Control of hemorrhage is a major concern and demands priority over attention to other injuries. After resuscitation and control of volume losses, efforts become necessary to minimize the potentially lethal postshock sequelae, including acute respiratory distress syndrome (ARDS) and multiple organ dysfunction syndrome (MODS).
Neurogenic Shock
Neurogenic shock is defined as failure of the nervous system to provide effective peripheral vascular resistance, resulting in inadequate end-organ perfusion. Warm, flushed, flaccid extremities; paraplegia; confusion; oliguria; and hypotension are the classic clinical findings. Injury to the proximal spinal cord, with interruption of the autonomic sympathetic vasomotor pathways, disrupts basal vasoconstrictor tone to peripheral veins and arterioles. Profound vasodilation of all microvascular beds below the level of cord injury diminishes venous return to the heart, reduces cardiac output, and precipitates hypotension. Injuries at or above the fourth thoracic vertebrae may disrupt sympathetic enervation to the heart, resulting in significant bradycardia and severe decompensation.
Similar to the initial therapy for shock resulting from hypovolemia, treatment of the relative hypovolemia due to vasodilation of neurogenic shock requires intravenous volume resuscitation. Restoration of the pathologically expanded intravascular space improves preload and cardiac output and may reverse hypotension. However, maintenance of adequate hemodynamics often requires vasopressor support in an effort to avoid the administration of excessive fluids. CVP monitoring to assess cardiac preload should be considered as a means of determining adequate and nonexcessive filling pressures, as loss of vasomotor capacity within the pulmonary circulation predisposes these patients to pulmonary edema. As spinal cord injury is often associated with other traumatic injuries, the diagnosis of isolated neurogenic shock must be a process of exclusion.
This condition should not be confused with spinal shock. Spinal shock is defined as a loss of sensation accompanied by motor paralysis with initial loss but gradual recovery of spinal reflexes following spinal cord injury. The reflexes caudal to the spinal cord injury are hyporeflexic or absent, while those rostral are unaffected. No circulatory compromise is associated with this condition; thus, it should not be considered a shock state.
Hypoadrenal Shock
The role of adrenocortical hormones in providing resistance to shock is well recognized. The reduction in effective blood volume and changes in blood chemistry that occur after adrenalectomy are similar to those of shock and hemorrhage. Adrenalectomized animals have markedly diminished tolerance to both trauma and hypovolemia. Adrenal cortical hormones also play a key role in maintaining normal capillary tone and permeability. In recent years, the concept of functional or relative adrenal insufficiency has received increasing attention as a cause of unrecognized shock and hypoperfusion. Most critically ill patients have elevated cortisol levels, but some have low concentrations in relation to the degree of stress imposed by their disease. Administration of physiologic doses of steroids to correct this insufficiency may result in stabilization of hemodynamics and possible survival benefits.38 However, the concept of routine administration of physiologic doses of steroids has been questioned; thus, routine and indiscriminate use is not recommended.39
Diagnosis of hypoadrenal shock is difficult, as classic signs of Addison disease are absent. The only clinical clues may be unexplained hypotension and refractory response to high-dose vasopressors. An isolated serum cortisol level is difficult to interpret because the range of values observed in critically ill patients varies considerably. A cortisol level below 15 μg/dL suggests a high likelihood of adrenal insufficiency, whereas a value above 35 μg/dL suggests adequate adrenal function.40 The adrenocorticotropic hormone (ACTH) stimulation test may be used to identify hypoadrenal patients when the diagnosis is unclear, but the utility of this test, particularly with an elevated baseline value, is of questionable utility.
The utility of the ACTH stimulation test is especially questionable in patients with persistent evidence of shock and elevated baseline levels of cortisol above 35 μg/dL. These patients actually demonstrate evidence of inadequate systemic cortisol utilization with a significant risk of morality, and thus may actually benefit from systemic administration of physiologic concentrations of corticosteroids.41
Although hypoadrenal shock may complicate various types of shock, there is conflicting evidence to support the use of supplemental corticosteroids in patients with septic shock if there is biochemical evidence of hypoadrenalism. Thus, supplemental corticosteroids should be used with extreme caution until further evidence is available.32,41
Physiologic Response to Hypovolemia
Common to each shock state is usually an initial decrease in circulating intravascular volume. This reduction is due directly to fluid loss or secondarily to fluid redistribution. This reduction in circulating fluid volume initiates both a rapid and sustained compensatory response. Within minutes, a rapid compensatory response occurs primarily due to adrenergic output. Sustained responses, in contrast, occur slower and result in intravascular fluid reabsorption and renal conservation of water and electrolytes (Algorithm 9-1).
Rapid Response
Hypovolemia results in the initial secretion of epinephrine and norepinephrine from the adrenal gland due to decreased afferent impulses from arterial baroreceptors. Catecholamine release is acute and limited to the first 24 hours following the onset of hypovolemia. This results in vasoconstriction, tachycardia, and increased myocardial contractility. Adrenergic-induced vasoconstriction of the systemic capacitance of small veins and venules shifts blood back to the central venous circulation, thus increasing right-sided filling pressures. Left-sided filling and pressure are augmented by pulmonary vasoconstriction. Concomitantly, vasoconstriction occurs in the skin, kidneys, and viscera, effectively shunting blood to the heart and brain. Adrenergic-induced vasoconstriction increases cardiac filling and causes increased contractility and reflex tachycardia, all of which combine to increase stroke volume and cardiac output.
Algorithm 9-1. Neurohormonal response to hypovolemia. CNS, central nervous system; ACTH, adrenocorticotropic hormone; AVP, arginine vasopressin.
Adrenergic-mediated vasoconstriction affects arterioles, pre- and postcapillary sphincters, and small veins and venules. Due to this specific vasoconstriction, decreased hydrostatic pressure distal to the precapillary sphincter occurs that leads to reabsorption of interstitial fluid (water, sodium [Na+], and chloride [Cl−]) into the vascular space. This functions to restore circulating blood volume and is known as transcapillary refill.42
Sustained Response
Sustained compensatory responses include the release of vasoactive hormones and fluid shifts from the interstitium and the intracellular space to the intravascular compartment. Decreased renal blood flow, increased adrenergic activity, and compositional changes in tubular fluid lead to the secretion of renin from the juxtaglomerular complex. Renin results in increased formation and release of angiotensin I by the liver. Circulating angiotensin I is rapidly converted to angiotensin II by the lungs and is the most potent known arterial and arteriolar vasoconstrictor. Angiotensin II also stimulates the release of pituitary ACTH. Increased circulating levels of both angiotensin II and ACTH result in increased secretion of aldosterone. As a result, reabsorption of Na+ in the distal renal tubules in exchange for potassium (K+) and hydrogen ions occurs.
Additionally, due to hypovolemia and increased serum osmolarity, reduced stimulation of arterial baroreceptors occurs, leading to the release of arginine vasopressin (AVP), also known as antidiuretic hormone (ADH). This hormone not only functions as a potent vasoconstrictor but also causes increased reabsorption of water by increasing water permeability and passive Na+ transport in the distal renal tubule. The overall net effect of aldosterone and AVP is to decrease glomerular filtration and increase salt and water tubular reabsorption in an effort to replace circulating intravascular volume deficits.
Finally, the increased release of the stress hormones (epinephrine, ACTH, cortisol, and glucagon) leads to glycogenolysis, lipolysis, and protein catabolism, causing a negative nitrogen balance and high extracellular concentration of glucose due to decreased insulin release and resistance. This leads to increased glucose utilization by insulin-independent tissues such as the brain and heart. In addition to glucose, products of anaerobic metabolism from hypoperfused cells accumulate in the extracellular compartment, inducing hyperosmolarity. This extracellular hyperosmolarity draws water from the intracellular space, increasing interstitial osmotic pressure, which in turn drives water, Na+, and Cl− across the capillary endothelium into the vascular space.
ORGAN-SPECIFIC COMPENSATORY RESPONSES TO SHOCK
Cardiac and Microvascular Response
Cardiovascular physiology is profoundly affected by shock (Table 9-3). Reduced stroke volume is caused by an absolute or relative loss of preload. Intrinsic neuroendocrine and renal compensatory responses, along with additional intravenous fluid, are needed to increase ventricular end-diastolic volume. Restoration of adequate preload alone is often sufficient to return cardiac output to levels required to overcome peripheral perfusion deficits. However, some cases are complicated by acquired myocardial contractility derangements. Contractile function under these conditions is less a function of preload and more related to intrinsic myocardial dysfunction.
A defining characteristic of shock is compromise of microvascular perfusion. Far from being a passive conduit, the microvasculature actively participates in the response to shock. Arteriolar vessels are innervated by sympathetic nerves, as are small veins and venules. The vasoconstriction of hypovolemic shock and the vasodilation of septic and neurogenic shock are a result of these autonomic responses. The majority of the circulating blood volume resides in the venous system, and normal physiologic compensation mechanisms rely on this venous blood pool as an autotransfusion reservoir. Collapse of underperfused veins passively propels blood toward the heart, while α-adrenergic venoconstriction actively mobilizes the venous pool. Profound peripheral vasoconstriction via α-adrenergic, vasopressin, angiotensin II, and endothelin-1 stimulation of arteriolar and precapillary smooth muscle sphincters selectively diminishes perfusion to dermal, renal, muscle, and, significantly, splanchnic vascular beds to preserve perfusion of critical central organs, primarily the central nervous system (CNS) and myocardium.43
The capillary endothelial monolayer maintains a semipermeable barrier between the intra- and extraluminal spaces and is compromised by shock.44 Circulating inflammatory mediators and byproducts of infection (LPS, thrombin, tumor necrosis factor alpha [TNF-α], interleukin [IL]-1, nitric oxide, and endothelin-1) generated in response to traumatic or septic shock have been shown to induce and sustain endothelial capillary leak. Although the exact mechanisms of endothelial monolayer dysfunction are unclear, the only available therapies to reverse microvascular decompensation are timely restoration of peripheral perfusion, rapid elimination of infectious and necrotic tissue, and pharmacologic and mechanical support of cardiopulmonary function.45–46
Neuroendocrine Response
The neuroendocrine reaction to shock consists of involuntary responses by the hypothalamus, autonomic nervous system, and secretory endocrine glands and is directed toward restoration of tissue perfusion and a redirected utilization of metabolic substrates. The autonomic response is initially triggered by hypoxia, hypotension, or hypovolemia detected by aortic and carotid baroreceptors and chemoreceptors (Algorithm 9-2). Subsequently, sympathetic vasoconstriction of specific vascular beds, induced by direct synaptic release of norepinephrine, results in redistribution of circulating blood volume from tissue of low metabolic activity to more metabolically demanding organs. Cardiac output, diminished by loss of preload, is augmented by inhibition of cardiac vagal activity and a resulting reflex tachycardia.
Circulating epinephrine and norepinephrine alter several aspects of glucose utilization, availability, and metabolism. The hyperglycemia of stress results from catecholamine-induced glycogenolysis, gluconeogenesis, and decreased pancreatic insulin release. Simultaneously, hypothalamic stimulation of the anterior pituitary induces release of ACTH, which in turn prompts cortisol and aldosterone release by the adrenal cortex. Elevated serum cortisol contributes to postinjury hyperglycemia by increasing gluconeogenesis, enhancing lipolysis, and diminishing peripheral utilization of glucose and amino acids. The pancreatic response is characterized by a decrease in insulin release and an increase in glucagon secretion, which further stimulates hepatic gluconeogenesis. The combined actions of catecholamines, cortisol, and glucagon are synergistic and create a shock-related hyperglycemia that is often refractory to insulin treatment.
Renal juxtaglomerular secretion of renin in response toadrenergic stimulation and renal hypoperfusion triggers the formation of angiotensin I in the liver, which is subsequently converted to angiotensin II by the lungs. Angiotensin II, a potent vasoconstrictor, augments shock-induced catecholamine-mediated peripheral and splanchnic vasoconstriction and stimulates aldosterone release from the adrenal cortex. Renal tubular reabsorption of sodium in response to elevated circulating aldosterone creates highly concentrated, low-volume urine. Vasopressin secretion by the posterior pituitary similarly contributes to compensatory restoration and maintenance of intravascular volume by promoting water reabsorption by the renal distal tubules and by causing peripheral and splanchnic vasoconstriction. A major result is a prolonged severe hypoperfusion of the splanchnic vascular bed, further augmenting the deleterious host response.
Algorithm 9-2. Shock resuscitation algorithm. ATLS, advanced trauma life support; ACLS, advanced cardiac life support; SBP, systolic blood pressure; CVP, central venous pressure; HCT, hematocrit; VS, vital signs; TTE, transthoracic echocardiography; PAC, pulmonary artery catheter; CI, cardiac index; MAP, mean arterial pressure.
Immunoinflammatory Response
Many shock-inducing events, particularly those associated with septic or traumatic shock, simultaneously trigger a massive systemic inflammatory response. Although inflammatory mediators (TNF-α, ILs, chemokines, etc.) play an integral role in the recovery of local tissue to trauma and infection, an uncontrolled systemic inflammatory response contributes to organ failure. Immunologically active cells involved in the systemic inflammatory response include nucleated blood cells (monocytes, polymorphonuclear leukocytes [PMNs]) and platelets, microvascular endothelial cells, and tissue macrophages. These cells generate and secrete scores of signal-amplifying inflammatory mediators ranging in complexity from individual molecules (nitric oxide) to large multisubunit, extensively modified proteins (TNF-α, IL-1, etc.). Even transient systemic elevation of any of these mediators has profound physiologic consequences.
Local tissue destruction, microbial contamination, and infection similarly activate the coagulation cascade and induce platelet aggregation and release of numerous platelet-, endothelial-, and clot-derived vasoactive mediators. Persistent, profound, and recurring microvascular hypoperfusion of the splanchnic and other organs likewise causes local tissue ischemia, parenchymal cell injury, microvascular coagulation, activation of inflammatory cells, and release of inflammatory mediators. Circulating monocyte, lymphocyte, and PMN adherence to activated endothelium results in extraluminal transmigration of these inflammatory cells into tissues remote from the area of injury. This hyperdynamic immunologic response, with continued generation of proinflammatory mediators, is responsible for the progression of SIRS toward MODS.47–50
Regulation of the systemic inflammatory response has been an active area of shock research for several decades. Elimination of particular inflammatory mediators from the systemic circulation, or inhibition of particular cell–cell interactions in experimental animal systems, has been shown to improve outcomes after shock resuscitation.51–55 However, to date, all efforts to regulate the SIRS response with therapeutic elimination or supplementation of specific proinflammatory and anti-inflammatory mediators have proven ineffective or even harmful in humans. This lack of efficacy may relate to inadequacy of current animal models of shock, sepsis, and organ failure or to inadequate understanding of the full spectrum and interactive nature of key elements that regulate this syndrome. Unfortunately, the network of inflammatory mediators and affected cells is so complex and redundant that no single agent is likely to be effective at interrupting this response. As a result, there is probably no single common pathway likely to respond to a “silver bullet” approach.
Pulmonary Response
The lung is the organ system most sensitive to systemic insult and injury and is frequently the first organ to fail in the progression to MODS. ARDS represents a high-mortality form of pulmonary insufficiency that is often precipitated by sepsis or traumatic shock. It is triggered and perpetuated by the numerous inflammatory mediators created in the hypoperfused microvasculature elsewhere in the body.51–56
Interstitial edema and subsequent reduced compliance result in diminished tidal volume and tachypnea, compromising gas exchange. Surfactant abnormalities contribute to alveolar collapse, resulting in loss of functional residual capacity (FRC) and the onset of pulmonary insufficiency. The pulmonary microvascular response mirrors the systemic response, with angiotensin and α-adrenergic–induced vasoconstriction creating significant elevations of pulmonary vascular resistance, further straining the heart. Pulmonary parenchymal injury may be propagated by excessive positive-pressure ventilation generated by alveolar overdistention in particular, further contributing to alveolar damage.
No specific measures are available to reverse the ARDS process; therefore, aggressive management of predisposing conditions is most appropriate. Once ARDS has become fully developed, treatment involves intensive supportive care while minimizing iatrogenic insults. As a result, early utilization of lung-protective strategies may diminish progressive ventilator-induced lung injury and improve outcome.57
Renal Response
Direct sympathetic-induced renal vasoconstriction increases afferent arteriole resistance in response to shock. This effect is reinforced by elevated circulating angiotensin II and catecholamines. The resulting decrease in renal blood flow and glomerular filtration rate (GFR), along with elevated circulating aldosterone and vasopressin (ADH), produce oliguria and prerenal azotemia. Acute tubular necrosis (ATN) may result from prolonged decreases in renal cortical blood flow, as well as from toxins generated during sepsis. Oliguric renal failure, like the pulmonary insufficiency of ARDS, is a common component of MODS.
Distinguishing low urine output due to oliguric renal failure from the oliguria of decreased renal perfusion pressure can be aided by analyzing urine sodium and osmolality. Renal hypoperfusion usually results in urine sodium of less than 20 mEq/L with an osmolality of greater than 400 mOsm/kg, whereas acute tubular injury impairs sodium reabsorption and is associated with a low urinary osmolality. A fractional excretion of sodium of less than 1% may also help determine whether the cause is renal or prerenal.
Early detection of abnormal kidney function is critical to prevent progression to renal injury. Abnormal renal function can be detected as a decline in creatinine clearance. The correlation between a creatinine clearance based on a 24-hour collection and a sample collected over a shorter interval is poor. However, the 24-hour value represents an average value over that time. A sample obtained over a shorter period may reflect the time in question and allow timely recognition and treatment of renal dysfunction.
Hepatic Response
Ischemic injury to liver is not apparent early during shock. However, the liver plays an important role in the regulation of shock and subsequent tissue injury through both the release of acute-phase reactants and lack of clearance of potential toxic agents. As shock continues, hepatic necrosis occurs, leading to the release of aspartate aminotransferase and alanine aminotransferase. Continued shock results in attenuated synthesis of coagulation factors, albumin and prealbumin. Although progressive ischemia can occur, leading to complete loss of glycogen stores and marked hypoglycemia, this condition is rare without pre-existing liver disease, significant direct liver injury, or hepatic artery occlusion.
Genetic Regulation of the Response to Shock
Individuals vary considerably in their susceptibility to shock and in their ability to recover from its consequences. Recently, unique host-specific responses have been identified as important determinants of the outcome of sepsis and septic shock. Genetic predispositions in an individual’s immunoinflammatory response may dictate whether infection is adequately or inadequately countered. These differences in response may be partially explained by single nucleotide polymorphisms (SNPs) in the genetic sequences for various inflammatory mediators. These subtle nucleotide variations may affect the transcription or translation of associated genes or the secretion or function of the corresponding proteins.
The recognition that genetic background may regulate the response to severe sepsis and septic shock has led to the identification of an array of genetic markers associated with enhanced risk of septic mortality. These SNPs are in genes encoding proteins involved in pathogen recognition (toll-like receptors 2, 4, and 5; CD14; and mannose-binding lectin), cytokine expression (TNF-α, IL-1, IL-6, and IL-10), and several other genes involved in mediating and controlling the innate immune response and the inflammatory cascade.58,59
Investigation into genetic polymorphisms should provide important insights into the pathophysiology of shock. SNP identification leading to early diagnosis of individuals at risk of developing or dying from the complications of shock may allow therapies to become more preemptive and effectively targeted.
LOSS OF COMPENSATORY RESPONSES TO SHOCK
If shock is prolonged, the arteriolar and precapillary sphincters become refractory and relax, while the postcapillary sphincter remains in spasm. Therefore, the capillary hydrostatic pressure increases, and Na+, Cl−, and water are moved into the interstitium, leading to depletion of intravascular volume.
Cellular membrane function is also impaired with prolonged shock. The normal negative membrane potential approaches neutrality, leading to increased permeability and interstitial flooding by K+. This is caused, at least in part, by a decrease in the normal function of the adenosine triphosphate–dependent Na+-K+ membrane pump that is a result of cellular hypoxia. The loss of the membrane potential difference leads to cellular swelling due to an intracellular influx of Na+ and fluid.
In addition to these cellular effects, severe and prolonged shock results in the development of progressive organ dysfunction. Although the mechanisms responsible are incompletely understood, it appears that the vital organs, in particular the lung early on, are limited in their ability to protect themselves during shock. As a result, direct endothelial injury occurs, resulting in leakage of various inflammatory mediators, which in turn results in accumulation of interstitial fluid and subsequent reduction in nutrient and gaseous diffusion. This condition, along with progressive microcirculatory thrombosis, is responsible for the late organ dysfunction characteristic of uncompensated shock.
COMPLICATIONS OF SHOCK
Ischemia–Reperfusion Injury
Inadequate microvasculature flow results in activation of leukocytes and converts local endothelial cells to a proinflammatory, prothrombotic phenotype. On reperfusion, the reintroduction of oxygen prompts these cells to generate superoxide anion, hydroxyl radicals, and hydrogen peroxide, further injuring local tissue. Microvascular endothelial adherence, monolayer transmigration, and local oxidative burst by activated neutrophils, along with the profound loss of endothelial monolayer integrity (i.e., microvascular capillary leak), contribute to massive interstitial edema after reperfusion. Although all tissues are sensitive to varying degrees, ischemia–reperfusion injury appears to be most detrimental to the pulmonary vasculature and the splanchnic circulations. Pulmonary interstitial edema and alveolar fluid accumulation are associated with the development of ARDS, whereas extensive visceral edema may contribute to development of ACS and mesenteric ischemia.
Second-hit Phenomena
Patients who have been successfully resuscitated from shock are at risk for what is referred to as the second-hit phenomenon. A single episode of severe or prolonged shock may precipitate organ failure, but, in addition, the initial insult may “prime” the inflammatory response, resulting in an augmented or prolonged response to a subsequent insult such as an infection, a second episode of blood loss, or major surgery.60 For example, after the primary event, circulating neutrophils demonstrate enhanced superoxide anion production, increased endothelial cell adherence, augmented cytokine response, and increased cytotoxicity. Not only do circulating innate immune cells demonstrate this priming effect, but tissue-fixed cells, in particular the macrophage, demonstrate altered phagocytosis and augmented cytokine release.61 This dysfunctional response leads to not only diminished microbial clearance and enhanced risk of nosocomial infection but also increased host tissue injury and subsequent development of MODS.
Multiple Organ Dysfunction Syndrome
6 MODS rarely develops as a result of isolated cardiogenic or neurogenic shock but is most often precipitated by septic and traumatic shock complicated by second-hit events such as repeated episodes of hypovolemia, subsequent infection, or repeated blood transfusions. Similarly, the presence of an unresolved inflammatory focus (such as devitalized necrotic tissue), undrained bodily fluids, or unresolved regional perfusion deficits can be the primary causes for the persistence of SIRS and the transition to MODS.
The effects of shock, resuscitation, and reperfusion and the subsequent development of MODS appear to depend on changes in the splanchnic and pulmonary microcirculations. These vascular beds appear to be major sites of the activation and subsequent immunoinflammatory mediator production that is responsible for the SIRS response. As splanchnic microcirculatory flow decreases in response to homeostatic vasoconstrictive responses during hypovolemia, excessive and prolonged hypoperfusion of the gut results in extensive microvascular injury and subsequent activation of endothelium, neutrophils, and macrophages. In addition, mucosal barrier disruption permits translocation of bacteria and bacterial toxins to circulate and reach the large tissue-fixed macrophage population in the liver. Extensive activation of the Kupffer cells in the liver results in the release of inflammatory mediators that cause distant organ injury through the systemic activation of other immune cells.
Not only is a proinflammatory phase of SIRS present, but there is also a compensatory anti-inflammatory phase of the SIRS response that is evolutionarily designed to counter the locally generated hyperimmune response and check systemic spread of proinflammatory mediators. If the compensatory anti-inflammatory response syndrome (CARS) results in excessive immunosuppression, it may contribute to susceptibility to primary and secondary infections that can then lead to organ failure.62
Numerous clinical trials utilizing proinflammatory and anti-inflammatory mediators as therapeutic interventions have been conducted. Unfortunately, few therapeutic agents designed to counter immune dysfunction have had significant impact on the prevention and treatment of MODS. Although studies are planned and undergoing, no study has demonstrated a sustained impact to date. Several potential targets, including interferon, have been suggested but this theoretical benefit may prove incorrect as so many other targets have in the past.63
Abdominal Compartment Syndrome
ACS is a highly morbid complication of reperfusion injury to the splanchnic viscera. This syndrome appears to be increasingly prominent due to aggressive resuscitation techniques that enable salvage of profoundly hypotensive and hypovolemic patients. Splanchnic ischemia and reperfusion result in extensive visceral capillary leak and interstitial edema of the bowel. Excessive volume resuscitation during this phase leads to grossly edematous viscera within the closed space of the abdomen, which dramatically increases intra-abdominal pressure, and compromises intra-abdominal organ function, increases renovascular resistance, limits diaphragmatic excursion, and may decrease cardiac output and elevate intracranial pressure. The clinical hallmarks of ACS are a distended and tense abdomen, diminished tidal volume, pulmonary edema, decreased cardiac output, oliguria, and elevated urinary bladder pressure. Presence of all or a major part of this syndrome should prompt consideration of an urgent operative laparotomy to decompress the abdomen and treat by leaving the abdomen open.
Hypothermia
Hypothermia (core temperature <35°C) is common during shock. In addition to immobilization, both prehospital and postadmission exposure can lead to conductive, convective, and evaporative heat loss, which should all be minimized. In addition, the administration of room temperature intravenous fluids and of cold-stored blood also contributes to hypothermia.64 Hypothermia increases fluid requirements and independently increases acute mortality rates.65
As the core temperature decreases, the rate of oxygen consumption also decreases, to approximately 50% of normal at 28°C. The decrease in oxygen consumption is accompanied by increased production of acid metabolites. A leftward shift in the oxyhemoglobin dissociation curve also occurs with hypothermia but is partially compensated by the acidosis. CNS effects progress from confusion and loss of manual dexterity to obtundation and frank coma as the core temperature decreases from 35°C to 26.5°C. The heart rate decreases to approximately half of baseline at 28°C, with a concomitant decrease in cardiac output. All cardiac electrical conduction intervals are prolonged, consistent with the changes in heart rate, and both atrioventricular dissociation and refractory ventricular fibrillation occur at 28°C. Other potential physiologic effects include ileus and pancreatitis (from cold enzyme activation) at temperatures lower than 35°C.
Compensatory responses to hypothermia include increased excretion of catecholamines, resulting in doubling of the basal metabolic rate, and increased production of thyroid hormones, further increasing the basal metabolic rate to five times baseline. Shivering can increase heat production as well, but it represents a significant energy expenditure and has been shown to be inhibited during episodes of hypotension or hypoxemia.66 Compensatory responses to hypothermia are lost at temperatures below 30°C or 31°C, and a state of complete poikilothermy is reached.
The treatment for hypothermia is rewarming. The core temperature should be obtained on admission of the trauma patient. Patients whose core temperatures are 33°C to 35°C can be treated with passive rewarming, warm blankets, and hot packs. Patients with core temperatures lower than 33°C require active rewarming. If the patient is unconscious, airway control should first be obtained. Because severe hypothermia causes vasoconstriction, noninvasive blood pressure measurements may not be feasible or accurate, and an arterial line should be placed for monitoring and blood gas sampling. The inspired gas through the ventilator should be heated to 41°C and fully saturated with water vapor to increase heat conductance in the lung. The intravenous fluids should also be warmed. Commercially available rapid infusion systems with countercurrent heating elements should be used. For extreme hypothermia, continuous mechanical arteriovenous rewarming can be performed for both circulatory support and rewarming. Recently developed microtechnology permits core rewarming by percutaneous placement of countercurrent warming coils directly in the inferior vena cava (IVC). Finally, other warming methods include lavage of heated saline through nasogastric and thoracostomy tubes as well as peritoneal lavage, but are not as effective.
Coagulopathy
Coagulopathy is a frequent problem complicating shock, especially in those patients who have received large volumes of crystalloid solution and blood for resuscitation. Although this problem is incompletely understood, it is clear that coagulation defects during shock are multifactorial. The presence of shock, the fluid volume required for resuscitation, the presence of hypothermia, and pre-existing diseases all influence the likelihood and severity of coagulopathy.67
A major factor in coagulopathy is usually due to the dilutional thrombocytopenia that occurs after massive volume resuscitation. Although bleeding times can be prolonged with platelet counts less than 100,000 cells/mL, platelet counts of 50,000 cells/mL or greater are usually adequate for surgical hemostasis. Dilutional thrombocytopenia becomes more likely with infusions of more than one blood volume. Each unit of platelets administered increases the platelet count by 10,000 to 15,000 cells/mL. Control of surgically remediable hemorrhage is prudent before platelet transfusion to prevent the loss of the transfused platelets.
Dilution of other coagulation factors also plays a role in development of coagulopathy. Factors V and VIII are the most labile in banked blood, but levels of less than 10% of normal for factors VII, X, XI, XII, and XIII are all associated with abnormalities in hemostasis, as demonstrated by prolonged partial thromboplastin time and prothrombin time. Fresh-frozen plasma can be administered as a source of all the soluble coagulation factors. The administration of cryoprecipitate may be necessary as a concentrated source of factor VIII and fibrinogen, particularly if adequate hemostasis is not obtained with the use of fresh-frozen plasma. Recent support has emerged for the use of recombinant activated factor VIIa. Although developed initially for use in hemophiliacs who developed inhibitors to factor VIII, anecdotal evidence has suggested that recombinant activated factor VIIa may serve to quickly reverse hemorrhage-induced coagulopathy.68 However, the use of factor VIIa is associated with significant complications including pulmonary embolism, myocardial infarction, and stroke and thus is no longer considered an optimal way to reverse hemorrhage-induced coagulopathy.69 Other agents have demonstrated promise in rapidly reversing coagulopathy, such as tranexamic acid for massive hemorrhage-induced coagulopathy and prothrombin concentrates for rapid reversal of anticoagulants.70–71
Finally, evidence has suggested that coagulopathy and hemorrhage can be minimized following massive blood loss if early aggressive use of fresh-frozen plasma is administered. Military data demonstrate that significant early coagulopathy is present after massive injury, even before blood component therapy is begun. Both civilian and military experience with a 1:1 ratio of packed red blood cells to fresh-frozen plasma has been associated with reduced mortality.72–74 However, this practice has been associated with an increase in the development of ARDS and organ dysfunction due to poorly defined mechanisms.75 Thus, generalization of this data to all patients with hemorrhage other than the massively injured should be performed with caution as demonstrated in the PROPRR study.76
TREATMENT
Fluid Therapy
Early investigators of hemorrhagic shock noted that decreased CVP and a reduction in total-body oxygen delivery (DO2) were key early findings. If the decrease in oxygen delivery was severe or prolonged, a reduction in total-body oxygen consumption ensued. After adequate fluid resuscitation, oxygen delivery and consumption increased above the baseline value for several hours, as if the body was paying back an “oxygen debt.” Failure of the patient to achieve this hyperdynamic response to resuscitation was almost always fatal. Because early death from shock appeared to be explained by the dynamics of oxygen delivery and utilization, therapy focused on restoring hemodynamics and oxygen transport with fluid and inotropes.
The provision of additional fluid beyond the amount of blood loss was associated with improved survival in both clinical and experimental studies of hemorrhagic shock, leading to widespread acceptance of aggressive fluid infusion. However, some researchers have recently described a significant increase in mortality associated with crystalloid overresuscitation and have postulated that excessive fluid administration increases the clinical risk of ARDS, MODS, increased intracranial pressure, and ACS.77–79 Because massive volumes of fluid are only provided to patients with severe shock, it is unclear if it is the excessive fluid or the associated underlying shock that increases the risk of ARDS, organ failure, or death after massive fluid resuscitation.
A minimum of two large-bore (14- to 16-gauge) intravenous catheters should be established in adults. Isotonic fluid is then infused at the same time as blood is obtained for arterial blood gas analysis, screening, and typing. Fluid can be infused up to 200 mL/min through a 14-gauge catheter and up to 220 mL/min through a 7-French catheter. A fluid challenge of 10 to 25 mL/kg is administered to the hypotensive patient and the response is assessed (i.e., 2,000 mL or 40% of blood volume of a 70-kg man). This therapeutic challenge is an effective trial in determining the amount of pre-existing or continuing volume loss. If the blood pressure returns to normal and is stabilized, the volume loss was relatively small, and the only treatment required may be infusion of isotonic fluid.
If the increase in blood pressure is transient after fluid bolus, then hemorrhage or continued fluid losses are severe and ongoing. Additional crystalloid is administered, and the need for blood transfusion is assessed. Patients who continue to require large amounts of fluid and blood to support perfusion usually have ongoing hemorrhage and require surgical intervention. No response or a minimal response to apparently adequate infusions of crystalloid solution and blood indicates exsanguinating hemorrhage and the need for urgent surgery.
Crystalloids
Balanced salt solutions are the most commonly used resuscitative fluids, and their use to restore extracellular volume significantly decreases the transfusion requirement after hemorrhagic shock. Lactated Ringer solution is isotonic, readily available, and inexpensive. It rapidly replaces the depleted interstitial fluid compartment and does not aggravate any pre-existing electrolyte abnormalities. Previous investigations have shown that administration of lactated Ringer solution does not lead to aggravation of the lactic acidosis that is present in shock.80 In fact, animal models have demonstrated that the use of blood plus lactated Ringer solution results in a more rapid return to normal lactate and pH than dose-shed blood alone. As volume and perfusion are restored, lactate is mobilized and metabolized to bicarbonate in a single pass through the liver. In fact, mild metabolic alkalosis may occur 1 or 2 days after large-volume resuscitations with lactated Ringer solution. Normal saline solution is also effective for resuscitation of hypovolemic patients. Concerns about inducing hypernatremic, hyperchloremic metabolic acidosis with massive resuscitation volumes remain but appear of less relevance by further investigation with normal saline and the hypertonic saline solutions.
Recently several investigations have raised concerns about the proinflammatory effects of resuscitation with lactated Ringer solution. Some commercially available lactated Ringer solutions contain racemic lactate that is made of equal concentrations of D(−)- and L(−)-isomers of lactate. The D(−)-isomer has been demonstrated in vitro to result in enhanced production of reactive oxygen species by neutrophils and inflammatory gene expression by leukocytes.81–82 In addition, increased apoptosis in both the small intestine and the liver was seen after resuscitation from hemorrhage with lactated Ringer solution but not with hypertonic solutions or blood resuscitation.83 These findings, however, are not consistent and do not appear dissimilar from saline resuscitation in other animal models.84 Thus, further investigations are required to determine the overall inflammatory effect of crystalloid resuscitation and extent of D(−)-isomer use in current commercial fluids.
Colloids
Colloids have the theoretic advantages of increasing the colloid oncotic pressure and requiring smaller volumes for resuscitation than crystalloids.85 Colloids commonly used for volume expansion in hypovolemia include albumin, dextran 70, dextran 40, and hydroxyethyl starch. Although each has unique individual characteristics, currently there is little justification for the routine addition of colloids to balanced salt solutions for volume replacement during shock. In fact routine use of colloid such as hydroxyethyl starch may be associated with increased risk of mortality without any benefit.86
Albumin solutions have been used during resuscitation to increase colloid oncotic pressure and, hypothetically, to protect the lung from interstitial edema; however, there is a relatively rapid flux of albumin across the pulmonary capillary membranes and relatively rapid clearance through the pulmonary lymphatics. In fact, colloid albumin infusion has been demonstrated to prolong the resuscitation phase and delay postresuscitation diuresis. Additionally, albumin may serve to depress circulating immunoglobulin levels and suppress albumin synthesis.
Dextran 40 and dextran 70 are polysaccharides with molecular weights of 40 and 70 kD, respectively. Dextran 40 (10%) is hyperoncotic and initially exerts a volume-expanding effect. However, because of its lower molecular weight, it is more rapidly excreted. Thus, dextran 40 is commonly used in cases of peripheral vascular disease and hyperviscosity syndromes. Dextran 70, conversely, is provided as a 6% solution and does not exert a hyperoncotic effect. The volume expansion is somewhat greater than the amount infused, and because of its large molecular size the effect is maintained for up to 48 hours. The dextran preparations, however, cause decreased platelet adhesiveness and decreased factor VIII activity. They also carry an incidence of allergic reaction of up to 5% and anaphylaxis of 0.6%.85,87
Hydroxyethyl starch is an amylopectin with volume-expanding effects for approximately 36 hours. It has side effects similar to those of dextran, but with less frequency. The incidence of anaphylaxis is 0.006%. A new hydroxyethyl starch, pentastarch, has a lower molecular weight and fewer hydroxyethyl groups than hydroxyethyl starch. Pentastarch has a shorter duration of action (2.5 hours) and has been reported to have even fewer side effects.87–88
The controversy regarding use of crystalloids versus colloids in resuscitation has not been resolved. Both types of solutions can restore circulating volume. The effects of the solutions on pulmonary function are at issue and are summarized as follows: (a) the use of crystalloid solutions decreases plasma oncotic pressure, thereby leading to lung edema at lower microvascular pressures; and (b) colloids given in the face of pulmonary injury can extravasate, promoting edema because of the reduced plasma interstitial oncotic gradient. In fact, a previous meta-analysis of colloid versus crystalloid resuscitation after hemorrhagic shock demonstrated a higher mortality rate among the colloid-resuscitated patients, partly because of pulmonary complications.89 Therefore, since colloid infusion has not demonstrated a significant benefit over crystalloid resuscitation alone, it is not currently recommended in the management of hypovolemic shock.90–91
Resuscitative Strategy
7 Aggressive fluid resuscitation is clearly a lifesaving modality and a key strategy in the treatment of shock and prevention of secondary consequences (Algorithm 9-2). However, indiscriminate fluid loading causes problematic edema in the lungs, gut, brain, and other organs. The amount of fluid used for resuscitation should be titrated to carefully selected hemodynamic and oxygen transport endpoints. Solutions currently in development (“artificial blood”) with oxygen transport capabilities may hold the potential of restoring oxygen transport while minimizing the need for large volumes.
Permissive Hypotension
Elevation of systemic arterial pressure in patients with disruption of the arterial system or major solid organ injury, especially after penetrating trauma, may cause acceleration of hemorrhage, disrupt natural clotting mechanisms, and cause dilution of clotting factors. Laboratory and clinical evidence support judicious use of intravenous fluids until hemorrhage is controlled by surgery, angiography, or direct pressure for penetrating trauma.92 Fluid resuscitation for patients with multisystem blunt trauma, especially with concomitant traumatic brain injury, represents a more complicated decision process, as maintaining cerebral perfusion pressure is a competing priority. Avoidance of both excessive fluid administration and prolonged hypoperfusion is best achieved in all patients not by maintenance of a marginal blood pressure, but by rapid surgical or angiographic intervention to control bleeding.
Transfusion
Anemia prompts clinical concerns because it may signify blood loss or hematologic disease, but it rarely causes tissue ischemia. The hemoglobin level that causes concern should depend on the adequacy of other mechanisms involved in oxygen delivery such as arterial oxygen saturation and cardiac output, the specific clinical situation, and the organ systems most at risk, balanced against the risk of transfusion. Clinical evidence suggests that hemoglobin values above 7 mg/dL are adequate in most patients, including the critically ill, but this has not been explored during shock. In one prospective randomized trial in critically ill patients, it was clearly demonstrated that a reduction in complications and improvement in survival were noted when lower hemoglobin values were accepted.93 However, this study excluded patients with hypovolemia, acute coronary syndrome, and sepsis. Although the role of transfusion during shock remains problematic, it appears in patients without shock these thresholds of transfusion are even acceptable for elderly patients with significant cardiac disease.94
Table 9-6 Comparison of Blood Availability
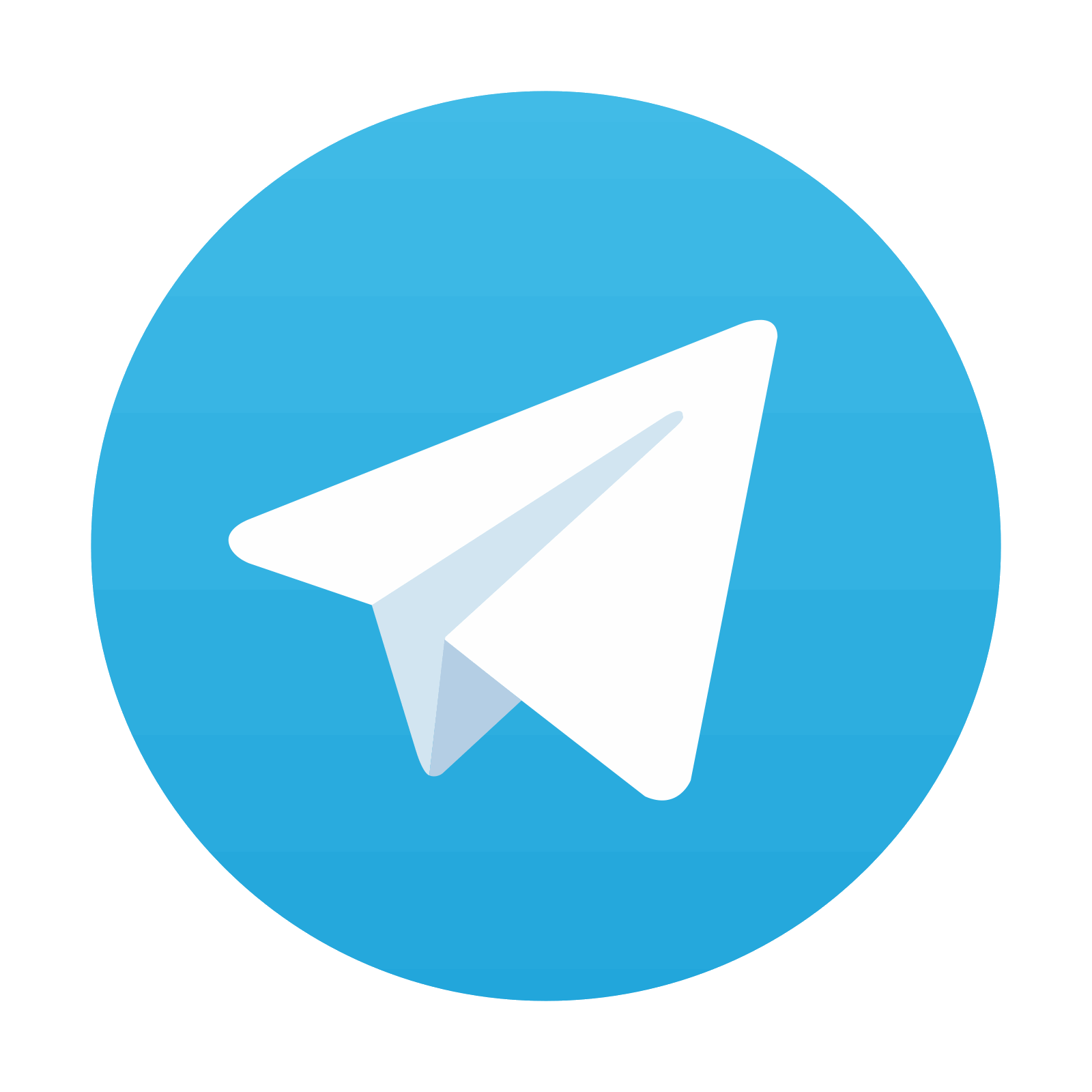
Stay updated, free articles. Join our Telegram channel
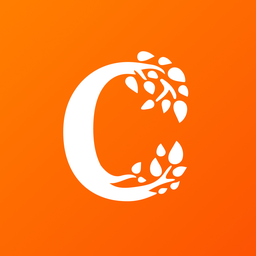
Full access? Get Clinical Tree
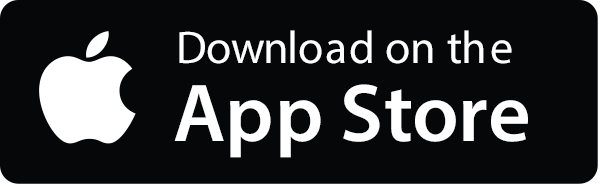
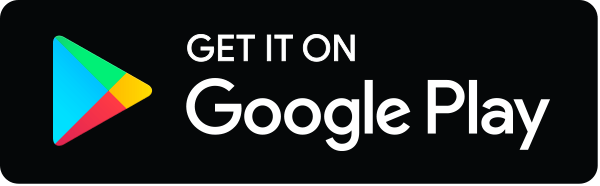