The rotavirus (RV) capsid is a nonenveloped triple-layered icosahedron that contains a genome of 11 segments of double-stranded (ds)RNA. In the cytoplasm, the transcription activity of partially uncoated RV particles produces capped (+)RNAs. These transcripts direct protein synthesis and act as templates for dsRNA synthesis. This replication pathway predicts that RV (+)RNAs are infectious when introduced into uninfected cells. Indeed, such is the case for the Reoviridae members: mammalian reovirus, bluetongue virus, African horse sickness virus, and epizootic hemorrhagic disease virus, where fully recombinant reverse genetics (RG) systems have been developed using viral (+)RNAs derived from T7 transcription vectors. Attempts to create similar fully recombinant RV RG systems have so far been unsuccessful. However, single-gene RG systems have been described that allow genetic manipulation of select RV genome segments. Here, we review the RV replication cycle and consider progress and challenges in the development of more powerful RG systems.
Rotavirus Replication and Reverse Genetics
Abstract
Keywords
1. Introduction
2. Rotavirus replication
2.1. Virus Structure
2.2. Viral Transcription
2.3. Viroplasm Formation and Function
2.4. Genome Replication and Particle Assembly

The VP4–VP7 outer capsid layer is lost from the RV triple-layered particle (TLP) during virus entry, yielding a double-layered particle (DLP). Within the cytosol, the DLP directs the synthesis of 11 types of capped (+)RNAs. These are translated, producing proteins that influence host cell processes, direct viroplasm formation, and support genome replication and virus assembly. In the viroplasm, viral (+)RNAs interact with the viral polymerase, VP1, and RNA-capping enzyme, VP3, and then undergo assortment. The core shell, consisting of VP2, encloses VP1-VP3-(+)RNA complexes, that, after forming core-like structures, support dsRNA synthesis. Newly formed cores interact with VP6 to form DLPs; these migrate to the endoplasmic reticulum (ER) due to the affinity of the DLP VP6 protein for ER-transmembrane protein, NSP4. During budding into the ER, assembly of the VP4–VP7 outer capsid layer around the DLP results in the formation of the TLP. PM, plasma membrane; ER, endoplasmic reticulum.
3. Reverse genetics systems
3.1. Fully Recombinant Systems
Table 2.3.1
Fully Recombinant Reverse Genetics Systems for the Reoviridae
Virus | Source of T7 RNA polymerase | Approach for generating recombinant virus | Key references |
Mammalian reovirus | Vaccinia virus rDIs-T7pol | Transfection of T7 transcription vectors (10-plasmid) | Kobayashi et al. (2007) |
BHK cell line expressing T7 RNA polymerase | Transfection of T7 transcription vectors (4-plasmid) | Kobayashi et al. (2010), Boehme et al. (2011) | |
Plasmid encoding T7 RNA polymerase | Transfection of T7 transcription vectors (10+1-plasmid) | Komoto et al. (2014) | |
Bluetongue virus | Cell-free T7 transcription system | Single transfection of synthetic viral (+)RNAs | Boyce et al. (2008) |
Cell-free T7 transcription system | Double transfection of synthetic viral (+)RNAs | Matsuo and Roy (2009) | |
Cell-free T7 transcription system | Sequential transfection of protein expression vectors and synthetic viral (+)RNAs | Matsuo and Roy (2013) | |
Cell-free T7 transcription system | Transfection of reconstituted cores containing in vitro replicated viral (+)RNAs | Lourenco and Roy (2011) | |
African horse sickness virus | Cell-free T7 transcription system | Double transfection of synthetic viral (+)RNAs | Matsuo et al. (2010), Kaname et al. (2013) |
Epizootic hemorrhagic disease virus | Cell-free T7 transcription system | Sequential transfection of protein expression vectors and synthetic viral (+)RNAs | Yang et al. (2015) |
3.1.1. MRV
3.1.2. Orbiviruses: BTV, AHDV, and EHDV
3.1.3. RV—Attempts at Developing Fully Recombinant RG Systems
3.2. Rotavirus Single-Gene Replacement Systems
Table 2.3.2
Single-Gene Reverse Genetics Systems for the Reoviridae
Virus | Target segment (protein) | Helper virus (species) | Source of RNA polymerase | Source of RNA transcripts | Selection and recovery of recombinant virus | Key references |
Rotavirus | g4 (VP4) | KU (human) | Vaccinia virus rDIs-T7pol | Transfection of g4 transcription vector | Passage with neutralizing αVP4 antibody | Komoto et al. (2006), Komoto et al. (2008) |
g7 (NSP3) | RF (bovine) | Vaccinia virus rDIs-T7pol | Transfection of g7 transcription vector | Serial passage at high MOI | Troupin et al. (2010) | |
g8 (NSP2) | SAlltsE (simian) | Vaccinia virus rDIs-T7pol | Transfection of g8 transcription vector | Passage in g8 shRNA-expressing cell line at non-permissive temperature | Trask et al. (2010), Navarro et al. (2013) | |
g10 (NSP4) | CHLY (bovine) | Plasmid encoding T7 RNA polymerase | Transfection of g10 transcription vector | Passage in g10 shRNA-expressing cell line | Yang et al. (2012) | |
Mammalian reovirus | S1 (σl) | T3D | Endogenous RNA pol II | Integrated S1 expression cassette | Binding of virion His-tagged s1 to cell-surface αHis-tag antibody | van den Wollenberg et al. (2008) |
S2 (σ2) | ST3 (ST2) | Cell-free T7 transcription system | Transfection of synthetic transcripts | Plaque assay | Roner and Joklik (2001) |
3.2.1. Gene 4 (VP4)
3.2.2. Gene 8 (NSP2)
Table 2.3.3
Recombinant SA11 Rotaviruses Made From the Temperature-Sensitive Mutant tsE (Navarro et al., 2013)
Recombinant virus | Features of the gene 8 RNA |
rSA11/g8SA11 | Represents an siRNA-resistant SA11 RNA |
rSA11/g8DC1 | Represents a DCl/SA11 chimera |
rSA11/g8DS-1 | Represents a DS-1/SA11 chimera |
rSA11/g8OSU | Represents an OSU/SA11 chimera |
rSA11/g8UK | Represents a UK/SA11 chimera |
rSA11/g8-3’PacI | Contains a Pac I site at the NSP2 ORF/3’-UTR junction |
rSA11/g8-3’25D | 3’-UTR contains a 25-nt sequence duplication |
rSA11/g8-3’50D | 3’-UTR contains a 50-nt sequence duplication |
rSA11/g8-3’100D | 3’-UTR contains a 100-nt sequence duplication |
rSA11/g8-3’200D | 3’-UTR contains a 200-nt sequence duplication |
rSA11/g8-3’Flag | 3’-UTR contains a nonexpressing Flag sequence |
rSA11/g8-3’HCV2 | 3’-UTR contains a nonexpressing HCV2-epitope sequence |
rSA11/g8-3’Flag-25D | 3’-UTR contains a nonexpressing Flag sequence and 25-nt g8 sequence duplication |
rSA11/g8-3’Flag-50D | 3’-UTR contains a nonexpressing Flag sequence and 50-nt g8 sequence duplication |
rSA11/g8-3’HCV2-25D | 3’-UTR contains a nonexpressing HCV2-epitope sequence and 25-nt g8 sequence duplication |
rSA11/g8-3’HCV2-50D | 3’-UTR contains a nonexpressing HCV2-epitope sequence and 50-nt g8 sequence duplication |
rSA11/g8-3’CrPV | 3’-UTR contains a CrPV IRES |
rSA11/g8-3’CrPV-Flag | 3’-UTR contains a FLAG expression sequence downstream of a CrPV IRES |
rSA11/g8-3’PP7 | 3’-UTR contains a recognition element for PP7 protein |
rSA11/g8-3’(2x)PP7 | 3’-UTR contains two tandem recognition elements for PP7 protein |
rSA11/g8-3’MS2 | 3’-UTR contains a recognition element for MS2 protein |
rSA11/g8-3’MS2-PP7 | 3’-UTR contains recognition elements for MS2 and PP7 proteins |
rSA11/g8-3’TAR | 3’-UTR contains HIV TAR element |
3.2.3. Gene 7 (NSP3)
3.2.4. Gene 10 (NSP4)
3.3. Summary and Future Directions
Acknowledgments
Note added in proof
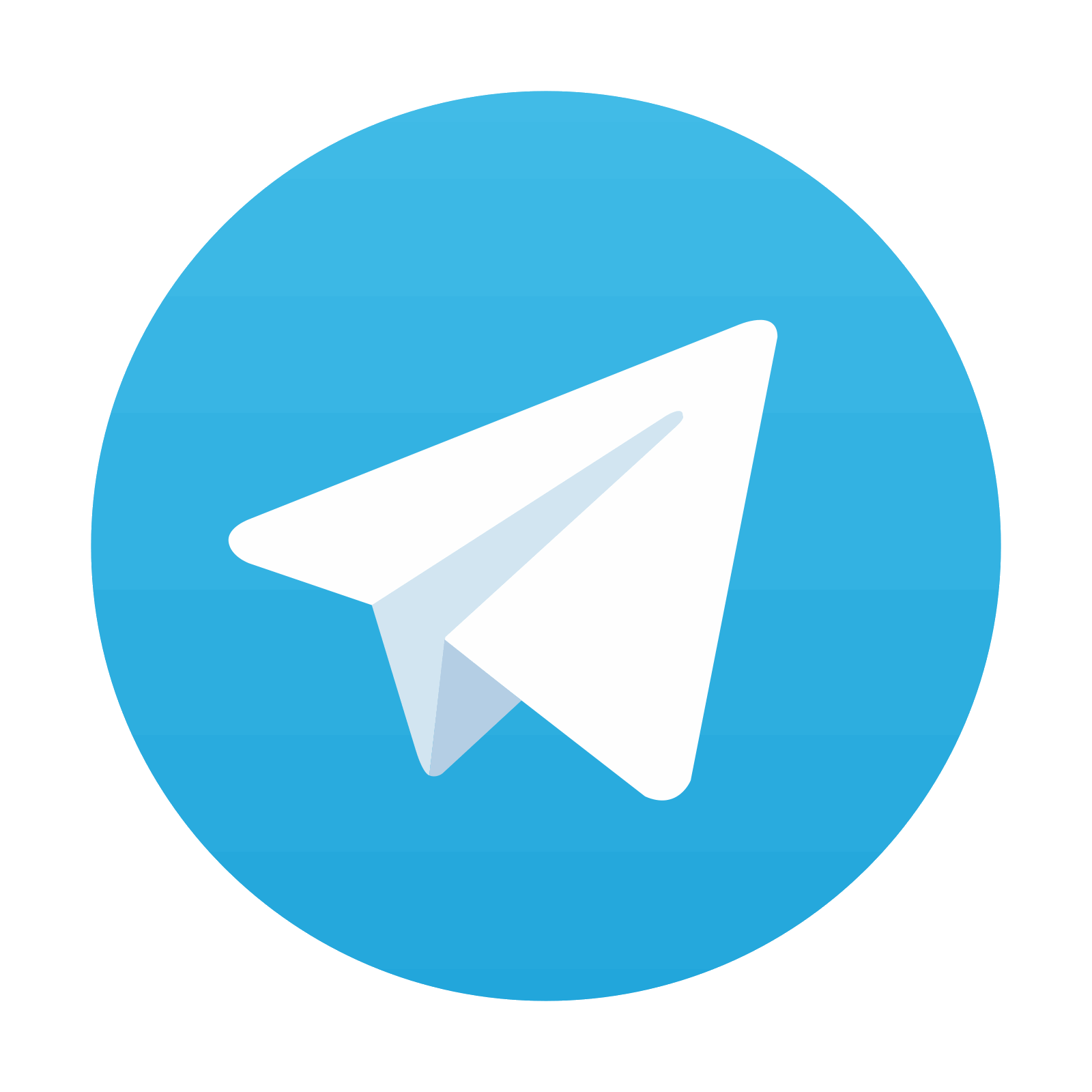
Stay updated, free articles. Join our Telegram channel
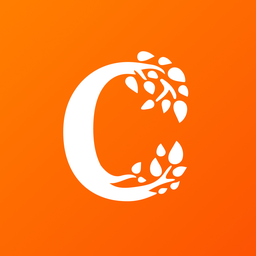
Full access? Get Clinical Tree
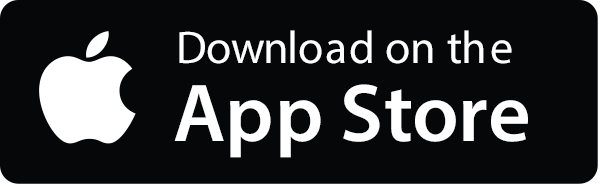
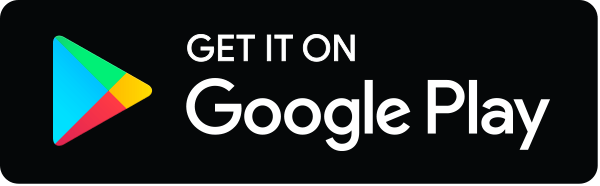