We review the basic classification of rotaviruses, as well as the mechanisms of rotavirus evolution and their significance. We then provide an overview of laboratory methods that have been used to characterize rotaviruses since their discovery in the 1970s, and the role that these methods have played in our understanding of the molecular epidemiology of rotaviruses. The global distribution of rotavirus genotypes, as well as, how these patterns vary over space and time, are described. Finally, we outline how our understanding of the transmission dynamics of rotavirus helps to explain the observed epidemiological trends.
Chapter 2.10
Molecular Epidemiology and Evolution of Rotaviruses
K. Bányai*
Abstract
Keywords
G type
P type
complete genotypes
reassortment
zoonotic
surveillance
transmission dynamics
1. Classification of rotaviruses
Rotaviruses form a genus of the Reoviridae family and possess a genome of 11 segments of double-stranded (ds) RNA (Estes and Kapikian, 2007). Currently at least eight rotavirus species, designated Rotavirus A to Rotavirus H and a tentative ninth species, Rotavirus I, are known. Rotavirus A (RVA) occurs in birds and mammals, RVB, RVC, RVE, RVH, and RVI have been detected in one or more mammalian hosts, whereas RVD, RVF, and RVG have been detected only in birds (Matthijnssens et al., 2012b; Mihalov-Kovács et al., 2015).
Classification below the virus species level includes the differentiation of serotypes, defined by the surface antigens, VP7 and VP4. Using the two antigen specificities, a dual nomenclature has been introduced in order to designate rotavirus strains. Serotypes of VP7 are designated with the letter G followed by a number. The antigenic variants of VP4 are classified into P serotypes (Estes and Kapikian, 2007). Strains belonging to other RV species have not been classified into serotypes because they are difficult to culture, preventing serological cross neutralization studies.
The recognition that antigenic features of both serotypes correlate with deduced amino acid sequences allowed the introduction of a genotyping system. The dual nomenclature was extended to include genotype numbers, which in the case of VP7 were identical to those of the serotype specificity, whereas in the case of VP4 some discrepancies were seen. Thus, if both genotype and serotype specificities are known for the VP4, the P type is often labeled to show both type designations, for example, P1A[8], P2A[6], P3[9], where numbers outside and inside the bracket, respectively, denote the serotype and genotype specificities (Estes and Kapikian, 2007). The number of genotypes of the surface antigens varies across rotavirus species. For example, to date at least 27 G types and 37 P types have been recognized within RVA, 21 G and 3 P types within RVB, and 10 G and 7 P types within RVC (Marthaler et al., 2012; 2013; Matthijnssens et al., 2011).
With the increasing availability of rotavirus sequence data, the genotyping system has been extended to all 11 genome segments permitting the empirical determination of cut-off values of genotypes within each gene (Matthijnssens et al., 2008b). In this new nomenclature the formula Gx-P[x]-Ix-Rx-Cx-Mx-Ax-Nx-Tx-Ex-Hx represents the genotypes of the VP7-VP4-VP6-VP1-VP2-VP3-NSP1-NSP2-NSP3-NSP4-NSP5-encoding gene segments, respectively, with “x’ indicating the numbers of the corresponding genotypes. Within RVA, at least 27 G, 37 P, 16 I, 9 R, 9 C, 8 M, 16 A, 9 N, 12 T, 15 E, and 11 H genotypes have been identified, with many new genotypes identified in the past few years. This scheme was found helpful to describe common genotype constellations in various hosts as well as to understand the origin and evolution of unusual strains (Matthijnssens et al., 2011). Currently, a robust genotyping system is available for RVA only. Analogous systems for RVB and RVC are being developed.
2. Mechanisms of rotavirus evolution and their significance
Mechanisms of rotavirus evolution are driven by the interplay among viral genes, adaptation to the host species, and escaping the host immune response.
2.1. Point Mutation
Point mutations in rotavirus genomes occur due to the error prone activity of the viral RNA polymerase. The mutation rate has been estimated to range around the value of 5 × 10−5 per nucleotide, meaning that roughly one mutation arises for every new copy of the rotavirus genome synthesized (Blackhall et al., 1996). The rate of evolution due to accumulation of point mutations over time have been found to vary by genes and genotypes in molecular evolution studies, showing values in the range of 1.1 × 10–3 to 8.7 × 10–4 nucleotide substitutions per site per year (Donker and Kirkwood, 2012; Matthijnssens et al., 2010).
The majority of mutations do not cause changes in the amino acid sequence and there is evidence of negative selection in the evolution of most genes (Donker and Kirkwood, 2012; Song and Hao, 2009). Genomic regions that tend to accumulate missense mutations include those encoding key epitopes on the surface of the neutralization antigens. Such mutations have been implicated in changes to affinity to antibodies and likely contribute to the antigenic drift of field strains due to immune selection.
2.2. Reassortment
Reassortment of segmented RNA viruses is a mechanism by which cognate genome segments are exchanged in progeny viruses upon infection of a single cell by two or more closely related virus strains. When these viruses contain different cogent genes, new genotype constellations may emerge. Reassortment of the surface antigens could result in a large number of antigen combinations. However, while over 80 G–P antigen combinations have been identified so far, only six globally common G–P combinations occur in human, globally most common strains, even though many G and P types are expressed by cocirculating strains (Fig. 2.10.1) (Dóró et al., 2014).

Figure 2.10.1 Summary of human G/P genotype combinations found to date.
Color codes: red, globally common; orange, regionally common and unusual; yellow, rare.
Color codes: red, globally common; orange, regionally common and unusual; yellow, rare.
The reason behind this phenomenon is unclear. One explanation operates with genetic or phenotypic incompatibility between some variants of the neutralizing antigen specificities (Iturriza-Gómara et al., 2001). An alternative scenario is that there is in vivo selection of combinations that result in masking of VP4 or VP7 neutralization epitopes. Strains hiding their neutralization epitopes after reassortment may evoke a weaker neutralizing antibody response, thus enhancing their fitness over less advantageous antigen combinations (Bányai et al., 2009).
2.3. Rearrangement
Rearrangement or intragenic recombination describes the insertions, deletions, and more commonly, gene duplications leading to sudden changes in the structure of genome segments. Normally, in gene duplications, the coding region of the template fragment remains unimpaired. Genome segments with duplicated fragments at the 3′ end are fairly common in rotavirus strains of some animal species as well as some atypical rotavirus strains shed by immuncompromised patients over prolonged periods (Desselberger, 1996). The evolutionary benefits from rearrangement are unclear, but may include more effective replication or an increase in protein coding capacity. Despite these putative advantages, strains with duplicated genes are very rare among those of many host species, including immunocompetent children.
2.4. Recombination
Several authors have reported evidence of both intratypic and intertypic recombination events in the genes encoding the VP7 protein (Parra et al., 2004; Phan et al., 2007). Similar to reassortment, the requirement beyond intergenic recombination between cognate genes of parental strains is the infection of a single cell by multiple rotavirus strains. However, additional details about the mechanisms are unknown. Theoretically, the result of recombination events could be the generation of new antigenic structures, which may help the new variants to escape the host immune response. However, a recent analysis suggests that intrasegmental recombination is rare among naturally cocirculating rotavirus strains and typically does not lead to onward transmission (Woods, 2015).
2.5. Interspecies Transmission
Initially, the distribution of rotaviruses was thought to be host-species restricted. More recent studies extending surveillance to many new geographic areas, including remote rural regions, and animal host species have revealed that animal strains play a key role in the genetic diversity of human rotavirus infections, primarily those caused by RVA strains.
There are two major routes by which heterologous rotaviruses may be acquired by a new host: (1) direct transmission of the rotavirus strain to a new host species and (2) reassortment between the rotavirus strains of any heterologous host species. Most interspecies direct transmission events lead to dead-end infection. Fully zoonotic strains are rarely detected in surveillance studies of human rotavirus infections (Bányai et al., 2012; Dóró et al., 2014). The low transmissibility in alternative hosts may be partially governed by host genetic factors or viral genes, for example, the one encoding NSP1 (see Chapter 2.8). Zoonotic transmission coupled by reassortment is a more efficient means for the introduction of new antigen specificities to which humans are immunologically naïve. An example is the introduction of G9 VP7 specificity during the 1990s from an animal, most likely the porcine, host (Iturriza-Gómara et al., 2000; Mijatovic-Rustempasic et al., 2011). Reassortants carrying a single or few animal-origin genes within a genetic background typical of human rotavirus strains may be more transmissible in the new host.
The zoonotic potential of RVAs has important implications for human rotavirus epidemiology. The diversity of rotaviruses in any host species, including humans, is readily refreshed by independent interspecies transmission events at any geographic location, coupled with global transmission of adapted strains. Thus, eradication or even elimination of rotavirus infections through vaccination and other human-targeted interventions is unlikely due to the large number of host species whose homologous rotaviruses and gene pools are viable in the human host.
3. Laboratory methods used to study the molecular epidemiology of rotaviruses
Understanding of the epidemiological features and evolutionary mechanisms of rotavirus infections has evolved over time along with improvements in laboratory methods. Early epidemiological studies relied on serotyping by MAb-EIA assays, RNA pattern analysis, and RNA-RNA hybridization, whereas today, most studies rely on multiplex genotyping reverse transcription-polymerase chain reactions (RT-PCRs) and more recently, on sequencing combined with phylogenetic analysis and other bioinformatics tools.
3.1. Serotyping and Subgrouping
Culturing rotaviruses has not become a routine laboratory method due to the varying isolation success of different rotavirus strains, although it was crucial in distinguishing serotypes (Wyatt et al., 1983). The findings that multiple serotype specificities exist prompted the realization that rotavirus vaccines would need to provide protection against an antigenically heterogeneous group of viruses. In routine laboratory procedures, serotype specificity has been determined by monoclonal antibody-based enzyme immunoassays (MAb-EIAs) targeting the VP7 (G serotypes) (Taniguchi et al., 1987). Large-scale surveillance studies conducted during the 1980s by MAb-EIAs uncovered the global distribution of four major VP7 serotypes of human rotaviruses, designated G1–G4 (Woods et al., 1992). In animal species, completely different serotype specificities were found to predominate, for example, G5 in pigs and serotypes G6 and G10 in cattle. In other cases, some overlap among the specificities of human and animal strains were observed, such as for G3 strains which prevailed in horses and dogs, but are also common in humans (Hoshino and Kapikian, 1994; Papp et al., 2013b,c).
The discovery of serotype-specific epitopes on the other surface antigen, VP4, was an important finding showing that the two surface antigens elicit neutralizing antibodies independently. However, this initially had little effect on routine epidemiological studies given that reliable MAb panels to distinguish VP4 (P) serotypes had not been developed. A limited number of studies using cross neutralization or cross-reactive MAbs in EIAs revealed that many human rotavirus strains carried the P1 (P1A and P1B) serotype specificity. Furthermore, some preferred combinations between G and P serotypes were observed: the G1, G3, and G4 VP7 specificities were more likely associated with P1A, whereas the G2 VP7 specificity showed a tendency to combine with the P1B VP4 gene (Estes and Kapikian, 2007). In animal rotavirus strains, a different distribution of VP4 serotypes (eg, P9 in pigs, and P6 to P8 in cattle) and surface antigen combinations were identified (Hoshino and Kapikian, 1994; Papp et al., 2013b,c).
Another set of MAbs developed against the VP6 protein of RVAs led to the recognition of antigenic variants of VP6 (Greenberg et al., 1983). Subgroup I strains were most commonly seen among animal RVAs and some human RVAs, whereas subgroup II strains were detected in most human and some porcine and lapine RVAs. A few other strains, mainly of animal origin, were characterized as [subgroup I + II] or [subgroup nonI, nonII] (Hoshino and Kapikian, 1994).
Altogether, studies using cross-neutralization assays and MAb-EIAs revealed differences in serotype and subgroup distribution among various host species.
3.2. Electropherotyping
From the 1980s onward, another arm of molecular epidemiology studies utilized RNA electropherotyping (also called RNA fingerprinting, RNA profiling, or RNA migration pattern analysis). The 11 segments of the rotavirus genome can be separated and visualized in polyacrylamide gels by silver staining (Herring et al., 1982). The relative migration of each segment in polyacrylamide gels has been found to be useful in diagnostic applications and can also be used to differentiate infections in local outbreak situations. Typically, RVA strains of mammalian hosts show a pattern of RNA bands in the 4-2-3-2 arrangement, while RVBs and RVCs are characterized by the 4-2-2-3 and 4-3-2-2 patterns, respectively (Saif and Jiang, 1994). Within RVAs, three major e-types were observed, including long, short, and super-short patterns. The difference lies in the relative migration of gene segment 11, which shows faster migration in long e-type strains and slower in short and super-short e-type strains. Within major e-types many additional variants occur; thus, in addition to the diagnostic benefits, the method has been found to be useful in molecular epidemiological studies demonstrating, for example, the changing prevalence of various e-types over time or permitting the tracking of viral spread in the community or during a hospital outbreak.
3.3. Whole Genome Hybridization
First insights into the genetic relationship among rotavirus strains were enabled by RNA–RNA hybridization studies. The number of hybridizing RNA bands between target RNAs and the isotope-labeled probe RNAs served as the basis to distinguish various genetic groups, referred to as genogroups. Among human rotaviruses, whole genome hybridization studies identified two major genogroups (Flores et al., 1982). The prototype strains were Wa (a serotype G1P1A[8] strain) and DS1 (a serotype G2P1B[4] strain). Molecular analysis of the medically important human strains revealed that the Wa-like genogroup mainly included long e-type strains that express the P1A VP4 and one of the common VP7 genes (G1, G3, and G4), whereas DS1-like strains included primarily short e-type strains that carry the P1B VP4 and G2 VP7 genes. Intergenogroup reassortants between Wa- and DS1-like strains have been identified, but are rare (Ward et al., 1990). Whole genome hybridization studies of animal strains revealed a limited relationship between strains detected in heterologous host species, including humans. On the other hand, RNA–RNA hybridization was the first method to firmly demonstrate that some unusual human strains (or parts of their genome) may originate from animals indicating the zoonotic potential of rotaviruses (Nakagomi and Nakagomi, 1996).
3.4. Multiplex Genotyping PCR and Sequencing
From the early 1990s, soon after the first rotavirus gene sequence data became available, a new RT-PCR-based method has been adopted for strain surveillance (Gentsch et al., 1992; Gouvea et al., 1990). This was corroborated by the finding that genotypes correspond closely to serotypes. Nested multiplex RT-PCR assays revolutionized routine surveillance and have become the method of choice recommended by the World Health Organization (WHO) in the generic protocol for hospital-based surveillance (WHO 2002; WHO 2009). Nested multiplex RT-PCR assays permitted genotyping of RVA strains based on the lengths of amplified DNA fragments that could be readily analyzed in agarose gels. This was the main method used for the genetic characterization of >150,000 human rotavirus strains in >100 countries worldwide (Bányai et al., 2012; Dóró et al., 2014), and has been adapted to both G and P type specificities. The flexibility of these RT-PCR-based methods permits rapid replacement of old typing primers or addition of new primers targeting emerging type specificities (Iturriza-Gómara et al., 2004).
A significant reduction in costs of traditional (ie, Sanger) nucleotide sequencing has opened new avenues in rotavirus strain typing. Over the past 30 years, >40,000 nucleotide sequences of rotaviruses have been deposited in GenBank, primarily for RVAs. Nearly 30,000 sequences were deposited within the past 5 years, showing a very strong motivation to understand the evolution of RV strains in the postvaccine marketing era. Sequencing and phylogenetic analysis have uncovered new type specificities and significant diversity within rotavirus genotypes. In large-scale sequencing and phylogenetic analyses, several genetic lineages and sublineages could be distinguished within most, if not all, G and P type specificities. Furthermore, classification of rotaviruses into lineages and sublineages typically permits the distinction of strains collected from various host species.
3.5. Whole Genome Sequencing
Although not routine yet, whole genome based strain characterization has become available worldwide to supplement traditional technologies. At present, over 1000 whole genome sequences are available for RVA, and a few dozens for RVB to RVI.
Assessment of rotavirus evolution and molecular epidemiology has been made possible by some large-scale whole genome based studies. These studies clearly demonstrated the relative conservation in the genotype constellations of backbone genes (ie, other than VP7 and VP4) of medically important RVA strains. Reassortment among cocirculating strains belonging to identical genotype constellations are fairly common (Iturriza-Gómara et al., 2001) whereas reassortment events among strains belonging to different genotype constellations are typically rare (Esona et al., 2013; Mijatovic-Rustempasic et al., 2011).
Many whole genome based studies have focused on the diversity and evolutionary origin of unusual human rotavirus strains. These studies, together with the increasing amount of genome sequence information collected from animal host species, have permitted new insights into the evolution of some unusual genotype specificities, for example, the canine or feline origin of many human G3P[3] and G3P[9] strains. In addition, bats in Africa and China have been recognized to carry rotaviruses with both usual and unusual human genes. More recently, pigs have been identified as a major source and reservoir of strains infecting humans, showing a complex multidirectional transmission pattern between these two host species (Matthijnssens et al., 2008a; Papp et al., 2013a; Theuns et al., 2015).
Further improvements and cost reduction of high-throughput sequencing technology will facilitate whole genome based comparisons, determination of preferred genotype constellations, identification of vaccine-derived genes in field strains, and tracking of evolution of vaccine strains and other rotavirus strains in the postvaccination surveillance era (Bányai and Gentsch, 2014).
4. Trends in rotavirus strain prevalence in humans
Molecular epidemiology studies have revealed that the distribution of rotavirus strains varies over both space and time. Various RVA genotypes have been found to cocirculate in any given location and strong fluctuations in the distribution of the different genotypes can occur from year to year. The reasons for these fluctuations are not well understood, but can likely be attributed to slight differences in homotypic versus heterotypic host immunity, as well as stochastic effects acting on genetic variation produced through mutation and reassortment (Matthijnssens et al., 2012a).
4.1. Global Genotype Distribution
The majority of rotavirus gastroenteritis (RVGE) cases are caused by one of the five common genotypes—the Wa-like G1P[8], G3P[8], G4P[8], and G9P[8] strains or the DS-1-like G2P[4] strains. These five genotypes accounted for 75% of all strains genotyped between 1996 and 2007, prior to widespread rotavirus vaccination in most countries (Bányai et al., 2012). G8, found in combination with P[6], P[4] or P[8], is the sixth most common G-type, accounting for 0.9% of all strains globally and 3.3–9.1% of strains in Africa (Bányai et al., 2012; Sanchez-Padilla et al., 2009). Recently, G12 strains have emerged and spread across the globe (Matthijnssens et al., 2010) and can cause a considerable proportion of RVGE cases during a given season. G12 strains accounted for 1.3% of genotyped strains between 2003 and 2007 (Bányai et al., 2012) and are typically found in combination with P[8] or P[6], with a Wa-like or reassortant backbone. Nontypeable and mixed infections (consisting of more than one G- or P-type) account for 9.5% and 5.6% of all typed infections, respectively (Bányai et al., 2012). There is no difference in the severity of RVGE caused by the different genotypes.
G1P[8] is the most common genotype, accounting for 38% of all genotyped strains (Bányai et al., 2012). However, the relative importance of G1P[8] strains has been declining in recent years. This genotype accounted for 65% of strains in studies published between 1989 and 2004 (Santos and Hoshino, 2005). The reasons for this decline are not well understood.
4.2. Regional Genotype Patterns
In the Americas, Europe, and the Western Pacific region, the five common RV G-types (G1–G4 and G9) accounted for 91–92% of all strains during the period from 1996 to 2007, whereas these five G-types accounted for 82% of strains in the Eastern Mediterranean Region and 71 and 75% of strains in Southeast Asia and Africa, respectively. G1 was the most common G-type in all six regions, but prevalence varied from 28% in Southeast Asia to 52% of strains in Europe. G2 was the second most common G-type in the Eastern Mediterranean (14%), Southeast Asia (20%) and Africa (10%), while G9 was more common in the Americas (18%) and Europe (13%), and G3 was more common in the Western Pacific (25%) (Fig. 2.10.2). G8 accounted for 9% of strains in Africa, while G12 accounted for 4% of strains in Southeast Asia; but these G-types accounted for <1% of strains in other regions. Nontypeable (NT) and mixed strain infections were more common in Africa, Southeast Asia, and the Eastern Mediterranean than in the Americas, Europe, and the Western Pacific (Fig. 2.10.2).

Figure 2.10.2 Prevaccination genotype distributions by WHO region. (Source: Based on data from Bányai et al., 2012.)
We can only speculate about the driving forces underlying these regional differences in rotavirus genotype distribution. Improved genotyping methods and increased surveillance efforts have led to the contribution of data from more and more countries in recent years, including greater representation from low income countries, which tend to exhibit a greater diversity of genotypes. Zoonotic transmission is likely responsible for the majority of rare and NT strains. Likewise, G8 strains appear to be closely related to bovine RVA strains, and therefore should be more common in countries where humans and animals live in close proximity, or where transmission can occur from unimproved water sources. Furthermore, mixed infections and infection with reassortant strains are expected to be more common in countries with a higher rate of rotavirus transmission.
4.3. Local Genotype Patterns
Within a country, the distribution of RV genotypes in a given season can vary from one location to the next. For example, during Jul. 2003–Jun. 2004, in Australia, 86% of typed strains in Melbourne were G1, while G3 (56%) was the predominant G-type in Perth and G2 (77%) was the most common G-type in Alice Springs (Kirkwood et al., 2009). Similar geographic variation in the prevalence of different genotypes has been observed in countries, such as the United States (Gentsch et al., 2009), Brazil (Leite et al., 2008), and India (Kang et al., 2013).
4.4. Temporal Changes in Genotype Distributions
Rotavirus genotype distributions also change over time. While G1P[8] is the predominant genotype most years, particularly in high income countries, other genotypes can cause the majority of RVGE cases in any given season. For example, during the period between 1988 and 2006 in Budapest, Hungary, G1P[8] was the predominant genotype in 13 of the 18 seasons (Fig. 2.10.3) (Bányai et al., 2009). However, G2P[4] was the predominant genotype in 1996–97, while G9 strains were most common in 2002–03 and 2004–06 and G4P[8] was the predominant genotype in 2003–04. Furthermore, the predominant lineage within G1 changed in 6 out of 11 seasons with available sequence data in which G1P[8] was the predominant genotype (Bányai et al., 2009). Similar turnover of the multiple lineages within G1P[8], as well as of the other genotypes, has been observed (Arista et al., 2006; Giammanco et al., 2014; McDonald et al., 2009; Wu et al., 2014).

Figure 2.10.3 Annual genotype distribution in Budapest, Hungary, 1989–2006. (Source: Adapted from Bányai et al., 2009; Pitzer et al., 2011a.)
In settings with 10 or more years of continuous surveillance of RV genotypes, cycling of the predominant genotypes is observed. The period of oscillations in the prevalence of different genotypes has been found to vary between 3 and 11 (or more) years (Pitzer et al., 2011a). Therefore, at least 4 years of surveillance data are needed to reliably determine the underlying distribution of RV genotypes in any given location.
4.5. Local Persistence, Global Spread
Sequence data lend support for both local persistence of individual RV lineages and circulation of lineages on a global scale. In a given location, the same rotavirus lineage has been observed over multiple years, even in temperate countries where RVGE exhibits a distinct seasonality with very low incidence in the summer months (Arista et al., 2006; McDonald et al., 2012). This is suggestive of local persistence of the virus in the population, possibly through low levels of asymptomatic infection in adults and other partially immune subpopulations (Phillips et al., 2010).
Nevertheless, when RV strains collected from a single locale are compared to contemporary and archival sequences available for other regions of the world, the observed genetic diversity is similar (McDonald et al., 2012). The global spread of RV strains was particularly apparent following the recent emergence of G9 and G12 strains. In both cases, one particular lineage was responsible for the worldwide spread of these novel G-types (Matthijnssens et al., 2010). Within just over a decade, both of these strains had spread throughout the globe (Matthijnssens et al., 2009, 2010). However, larger than normal outbreaks of RVGE were not associated with the emergence of these new genotypes, which suggests that heterotypic immunity provides at least some protection against novel strains.
4.6. Impact of Vaccination on Genotype Distributions
Two rotavirus vaccines have been available since 2006, including the monovalent Rotarix® vaccine (GlaxoSmithKline Biologicals, Rixensart, and Belgium) and the pentavalent RotaTeq® vaccine (Merck and Co., Whitestation, NJ, and USA). These vaccines differ in their approach to eliciting immunity (Ward, 2009). The Rotarix vaccine consists of an attenuated Wa-like G1P[8] strain, whereas RotaTeq contains a mixture of 5 bovine-human monoreassortant RVA strains, where each strain contains either a human VP7 (G1, G2, G3, and G4) or VP4 (P1A[8]) gene, introduced by in vitro reassortment in the genetic background of the bovine WC3 rotavirus (G6P7[5]) strain. (See Chapter 2.11.)
Although there is a broad scientific consensus that both vaccines are effective in reducing the burden of severe RVGE, there is still controversy regarding the long-term effects of vaccination on the circulating RV genotype distribution (Matthijnssens et al., 2012a). Vaccine effectiveness has been found to be equally high against both homotypic and heterotypic strains (Leshem et al., 2014). Nevertheless, transient changes in the genotype distribution have been observed following large-scale vaccination with Rotarix and/or RotaTeq.
The introduction of RotaTeq was followed by an increase in the prevalence of the G3P[8] genotype in some places, including the United States and some Australian states (Hull et al., 2011; Kirkwood et al., 2011), raising concern that this could be linked to lower rates of seroconversion to the G3 component of the pentavalent vaccine (Matthijnssens et al., 2012a). However, similar observations have not persisted. Other genotypes, including G9P[8], G12P[8], and G2P[4], have predominated for a year or two in certain locations, but overall G1P[8] has remained the predominant genotype in countries using RotaTeq.
For Rotarix, a consistent and more prolonged increase in the relative prevalence of the fully heterotypic G2P[4] genotype has been observed following large-scale vaccination (Dóró et al., 2014), particularly in Brazil, Belgium, and some Australian states. However, the magnitude and duration of the increase varied between locations. In Australia, the increase G2P[4] was seen for two seasons following vaccine introduction (2007–09) and again in 2012, but G1P[8] and G3P[8] were the most common genotypes in 2009–11 and 2013, respectively, in states using Rotarix. A clear increase in G2P[4] after vaccine introduction has also been noted in Belgium, and has been sustained for at least seven seasons, 2006–13 (Zeller et al., 2010; Pitzer et al., 2015). In Brazil, G2P[4] has been the predominant genotype for at least six years since vaccine introduction (2006–11), with prevalence ≥50% in all years except 2009 (Gómez et al., 2014). Furthermore, G1P[8] has been relatively rare, having been detected in ≤20% of samples in all seasons since vaccine introduction in Brazil (Carvalho-Costa et al., 2011), while another fully heterotypic genotype (G8P[4]) emerged in Northeast Brazil in 2012 (Gurgel et al., 2014). The high prevalence of G2P[4] RVA strains in Brazil after introduction of the universal RV vaccination with Rotarix was not unique but also recorded in other South American countries where no RV vaccination program had been established (Matthijnssens et al., 2009).
Finally, a number of vaccine-derived reassortant strains have also been isolated from individuals both with and without a history of vaccination. In particular, vaccine-derived reassortant G1P[8] strains have been described in patients from the United States, Australia, and Finland following vaccination with RotaTeq. Reassortment between wild-type G1P[8] and the Rotarix vaccine strain has also occurred (Dóró et al., 2014). This has important implications for surveillance, as more advanced laboratory methods may be needed to differentiate between wild-type and vaccine-derived strains.
5. Rotavirus transmission dynamics: driving forces behind epidemiological trends
Rotavirus is transmitted via the fecal-oral route. Improvements in sanitation have had only a minor impact on the level of rotavirus transmission, unlike for the bacterial causes of diarrhea. The overall incidence of RVGE and the age distribution of cases do not differ much between developed and developing countries (Parashar et al., 2003). The vast majority of reported RVGE cases occur in children <5 years of age, who have little or no immunity. However, infection with rotavirus continues to occur throughout life. Most infections in adults are mild or asymptomatic, but may contribute to maintenance of transmission in the population (Anderson and Weber, 2004).
In countries with temperate climate, most cases of RVGE occur during winter epidemics. In tropical countries, rotavirus tends to be more common during the dry season, but RVGE cases typically occur throughout the year. However, the strength of rotavirus seasonality is more closely linked to the level of country development than it is to geographic location or climate (Patel et al., 2013). For instance, high-income countries in the tropics, such as Hong Kong, tend to experience seasonal outbreaks, whereas lower-income countries in temperate regions, such as Uzbekistan, exhibit year-round disease (Fig. 2.10.4).

Figure 2.10.4 Age distribution and seasonality of rotavirus in different countries.
The average number of RVGE cases per month (scaled relative to the maximum monthly number of RVGE cases) is plotted in blue; the average number of cases across all months is represented by the dashed black line. The proportion of cases in each age group (0–5 months, 6–11 months, 1–2 years, 2–3 years, 3–4 years, and 4–5 years old) is plotted according to the black bars for each country. Example countries are plotted by latitude (north to south) for (A) high-income countries and (B) developing countries. (Source: Adapted from Pitzer et al., 2011b.)
The average number of RVGE cases per month (scaled relative to the maximum monthly number of RVGE cases) is plotted in blue; the average number of cases across all months is represented by the dashed black line. The proportion of cases in each age group (0–5 months, 6–11 months, 1–2 years, 2–3 years, 3–4 years, and 4–5 years old) is plotted according to the black bars for each country. Example countries are plotted by latitude (north to south) for (A) high-income countries and (B) developing countries. (Source: Adapted from Pitzer et al., 2011b.)
Nearly all children have been infected with rotaviruses by the age of 2 years. The likelihood and severity of RVGE is associated with the number of previous rotavirus infections. Infants who have not been previously exposed to the virus are more likely to experience RVGE, which may be moderate or severe. Maternal immunity provides some protection against symptoms, particularly during the first few months of life. Following two natural infections, moderate to severe RVGE is rare in most children (Velázquez et al., 1996). However, in poorer countries where immunity may be compromised by factors, such as malnutrition, concomitant infections of the gut, and tropical enteropathy, severe RVGE can continue to occur following three or more natural infections (Gladstone et al., 2011). Death due to rotavirus is infrequent in most developed countries, but worldwide rotavirus was estimated to cause nearly half a million deaths each year prior to the introduction of universal vaccination programs, with most deaths occurring in developing countries (Tate et al., 2012).
Infants appear to be major drivers of transmission of rotavirus in the community. Vaccination has resulted in both direct protection for infants who received the vaccine, as well as indirect protection resulting from a reduction in the transmission of rotavirus in the population and (possibly) contact immunity gained from shedding and circulation of vaccine strains. Decreases in the incidence of RVGE following vaccine introduction have been observed in age groups not eligible for the vaccines (Lopman et al., 2011; Payne et al., 2011). Furthermore, changes to the timing and seasonality of rotavirus epidemics have been observed following vaccine introduction in some temperate countries, including delayed epidemics and a shift from annual to biennial (every other year) epidemics in the United States (Tate et al., 2013). These observations, combined with insights from mathematical modeling, suggest that by preventing RVGE in infants, vaccination also serves to decrease the transmission of rotavirus in the community.
5.1. What can Mathematical Models Tell us About Rotavirus Transmission?
Mathematical models can provide insight into the underlying population dynamics of rotavirus infections and important aspects of transmission. By analyzing the natural history, immunity, and transmission feedbacks of rotaviruses and describing these processes using a series of equations or mathematical relationships, models were established that allow one to bridge the gap between the individual course of infection and the population-level spread of disease.
A variety of mathematical models for rotavirus infections have been developed. These models vary in their structure and parameters based on different data sources and interpretations of the literature. Most models were developed with the aim of predicting the direct and indirect effects of vaccination. Different models predict similar reductions in RVGE in the first 5 years following vaccine introduction, but predictions for the long-term impact of vaccination have varied depending on assumptions about the relationship between age at infection and the severity and reporting of RVGE, as well as the duration of vaccine-induced immunity (Pitzer et al., 2012).
Models are also useful for explaining observed patterns of data. For instance, one model was able to demonstrate that the southwest-to-northeast pattern of rotavirus epidemics in the United States prior to vaccine introduction could be linked to underlying variation in the birth rate (Pitzer et al., 2009). Higher birth rates typical of states in the southwest lead to a quicker build-up of numbers of fully susceptible infants, who serve as important drivers of transmission. Models also predicted that rotavirus epidemics could become biennial at high levels of vaccine coverage, which has aided in the interpretation of patterns that have emerged since vaccine introduction.
Models have also attempted to explain the interaction among different RV genotypes, for example, modifying this in light of a couple of recent publications (e.g. Pitzer et al., 2011a). This model was able to show that the observed cycling of genotypes could be explained by differences in homotypic versus heterotypic host immunity. If immunity is stronger against a second infection with the same genotype as that causing the first infection (ie, if homotypic immunity is stronger than heterotypic immunity), then over time there will be a build-up of population-level immunity to the prevailing strain. This provides a selection pressure in favor of the rarer genotypes, allowing them to infect more people. Thus, over time one of the rarer genotypes is expected to increase in prevalence, while the prevailing genotype decreases in prevalence, and then the cycle repeats (Fig. 2.10.5).

Figure 2.10.5 Example output for strain-specific model of rotavirus transmission dynamics.
(A) The total number of severe RVGE cases per week (relative to the maximum number of weekly cases predicted by the model) is plotted for a 10-year period for model parameters typical of a high-income country (Pitzer et al., 2011a; Pitzer et al., 2015). (B) The mean annual proportion of severe RVGE cases caused by each strain, representing the five major G-types, is plotted for each year. (C) The average genotype distribution over the 10-year period.
(A) The total number of severe RVGE cases per week (relative to the maximum number of weekly cases predicted by the model) is plotted for a 10-year period for model parameters typical of a high-income country (Pitzer et al., 2011a; Pitzer et al., 2015). (B) The mean annual proportion of severe RVGE cases caused by each strain, representing the five major G-types, is plotted for each year. (C) The average genotype distribution over the 10-year period.
Models suggest that vaccination with a monovalent vaccine, such as Rotarix is expected to exert different selection pressures on the rotavirus population than vaccination with a multivalent vaccine, such as RotaTeq. This selection pressure can occur even in the absence of strain-specific variation in vaccine effectiveness against RVGE (Pitzer et al., 2015). If the relative risk of infection with a fully heterotypic strain (ie, G2P[4]) is greater than the risk of second infection with G1P[8] following vaccination with Rotarix, then this can explain the predominance of G2P[4] in the years following vaccine introduction in countries, such as Belgium. Nevertheless, both vaccines are estimated to provide a broadly heterotypic immunity in most fully vaccinated infants and to remain effective despite any possible changes to the genotype distributions (Pitzer et al., 2015). In order to understand and predict the impact of vaccination on the distribution of rotavirus genotypes, it is important to take into account differences in the risk of infection (and thus transmission), and not just differences in vaccine effectiveness against RVGE.
Mathematical models are only as good as the data that are used to inform them. Often it is necessary to make simplifying assumptions in order for the models to be tractable. Individual rotavirus strains are continually evolving over time through evolutionary processes, such as point mutations and reassortment, which can be difficult to represent in a mathematical model. Nevertheless, models provide a “test-bed” for examining hypotheses regarding how individual-level characteristics of infection can affect the population-level spread of disease, and can help to highlight important unknown factors of outbreak developments.
6. Conclusions
The diversity of rotavirus strains infecting both human and animals has been recognized since soon after their discovery in the early 1970s. Recent advances in laboratory methods for the detection and classification of rotaviruses have aided in the study and interpretation of epidemiological and evolutionary patterns. The recent introduction of vaccines has made the continued surveillance and development of new approaches to interpret data on the molecular epidemiology of rotavirus an imperative. Our understanding of rotavirus epidemiology and evolution will no doubt be tested and continue to be refined in the near future.
Acknowledgments
Financial support obtained from the Hungarian Scientific Research Fund (OTKA, T100727) (K.B.) and the US National Institutes of Health (R01 AI112970) (V.E.P.) is acknowledged. Fig. 2.10.1 was prepared and kindly shared by Renáta Dóró.
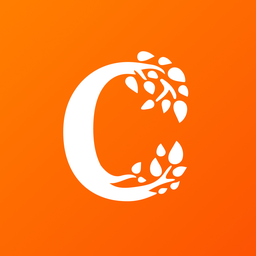
Full access? Get Clinical Tree
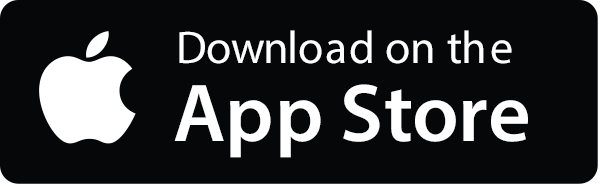
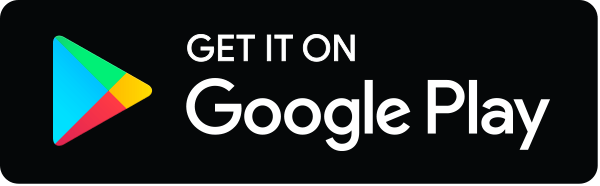