Noroviruses are a major cause of acute gastroenteritis and belong to the family Caliciviridae. Replication of the positive-sense single-stranded RNA genome is carried out by an error-prone RNA-dependent RNA polymerase (RdRp) that drives adaptation and evolution. The noroviruses are remarkably diverse, with seven major phylogenetic genogroups (G) each comprised of one or more genotypes. Among the human norovirus pathogens, multiple genotypes within GI, GII, and GIV, as well as variants of these genotypes, can be detected in epidemiologic surveys, with genotype GII.4 predominating globally as the major cause of infectious diarrheal disease. The marked diversity as well as the ability of strains to undergo recombination have led to the development of a unified dual nomenclature system that defines both RNA-dependent RNA polymerase (P) and capsid protein (C) genotypes. This chapter provides a summary of norovirus epidemiology and evolution, and ongoing efforts to translate this knowledge into the development of effective vaccines.
Molecular Epidemiology and Evolution of Noroviruses
Abstract
Keywords
1. Introduction
2. Classification and nomenclature of the noroviruses
2.1. Genus Norovirus in the Family Caliciviridae
Table 3.5.1
Taxonomic Structure of the Caliciviridae
Genus | Species | Representative strain |
Norovirus (NoV) | Norwalk virus (NV) | NoV/GI/Hu/US/1968/GI.P1-GI.1/Norwalk |
Sapovirus (SaV) | Sapporo virus (SV) | SaV/GI/Hu/JP/1982/GI.1/Sapporo |
Lagovirus (LaV) | Rabbit hemorrhagic disease virus (RHDV) European brown hare syndrome virus (EBHSV) | LaV/RHDV/Ra/DE/1988/GH LaV/EBHSV/Ha/FR/1989/GD |
Vesivirus (VeV) | Vesicular exanthema of swine virus (VESV) Feline calicivirus (FCV) | VeV/VESV/Po/US/1948/VESV-A48 VeV/FCV/Fe/US/1958/F9 |
Nebovirus (NeV) | Newbury-1 virus (NBV) | NeV/NBV/Bo/UK/1976/Newbury-1 |
The cryptograms in this table are organized as follows: genus/genogroup or virus species/host of origin/country of origin/year of occurrence/Pol genotype (if known)/Capsid genotype (if known)/strain name. Abbreviations for the host species are: Bo, bovine; Fe, feline; Ha, Hare; Hu, Human; Po, Porcine; Ra, Rabbit. Country abbreviations are: DE, Germany; FR, France; JP, Japan; UK, United Kingdom; US, United States. GenBank Accession numbers of representative viruses: NV, M87661; SV, U65427; RHDV, M67473, EBHSV, Z69620; VESV, AF181082; FCV, M86379; NBV, DQ013304.
2.2. Norovirus Genogroups and Genotypes

(A) Genome organization and nucleotide boundaries of the ORFs of representative human norovirus strains Genogroup I Norwalk virus (M87661) (top) and Genogroup II Lordsdale virus (X86557) (bottom). (B) Proteins encoded by the norovirus genome. ORF1 encodes the nonstructural proteins of the virus, NS1-NS7. ORF2 encodes the major capsid protein, VP1, and ORF3 encodes the minor capsid protein, VP2. (C) Genomic regions targeted for the norovirus dual genotyping system involve RNA-dependent RNA polymerase (P) and capsid (C) sequences. The regions of interest are amplified in a reverse transcription-polymerase chain reaction (RT-PCR) assay, sequenced, and then interrogated against a sequence database using the norovirus typing tool (Kroneman et al., 2013). References for representative primer sets are: (Anderson et al., 2001; Jiang et al., 1999b; Kojima et al., 2002; Newman and Leon, 2015; Vinje and Koopmans, 1996; Vinje et al., 2004). A widely-used real time RT-qPCR assay for norovirus detection is based on the primers of Kageyama et al. (Kageyama et al., 2003). Many additional primer pairs have been developed, but periodic optimization may be needed to encompass ever-evolving norovirus strains (Kong et al., 2015).
2.2.1. ORF1
2.2.2. ORF2
2.2.3. ORF3 (and ORF4)
2.2.4. Genotyping Methods
2.2.5. Genotyping System Based on the Viral Capsid

There are presently seven major genogroups within the genus Norovirus, with human pathogens found in GI, GII, and GIV. Porcine norovirus strains have been found also in GII, and canine and feline strains have been detected in GIV. Strains belonging to certain genogroups have not been detected in humans thus far and include GIII (bovine), GV (murine), and GVII (canine). Phylogenetic analyses were carried out with MEGA v6 using Neighbor-Joining as the algorithm for reconstruction and amino acid sequences from the entire capsid protein VP1. Scale bar represents the number of amino acids substitutions per site. (Source: Analysis and image courtesy of Gabriel I. Parra.)
Table 3.5.2
Norovirus Genogroups and Genotypes as Determined by Capsid (C) Gene Relatedness
Reference virus | Genogroup. C genotype | GenBank accession number |
GI/Hu/US/1968/GI.1/Norwalk | GI.1 | M87661 |
GI/Hu/UK/1991/GI.2/Southampton | GI.2 | L07418 |
GI/Hu/SA/1990/GI.3/DesertShield 395 | GI.3 | U04469 |
GI/Hu/JP/1987/GI.4/Chiba 407 | GI.4 | AB042808 |
GI/Hu/GB/1989/GI.5/Musgrove | GI.5 | AJ277614 |
GI/Hu/DE/1997/GI.6/BS5(Hesse3) | GI.6 | AF093797 |
GI/Hu/GB/1994/GI.7/Winchester | GI.7 | AJ277609 |
GI/Hu/US/2001/GI.8/Boxer | GI.8 | AF538679 |
GI/Hu/CA/2004/GI.9/Vancouver730 | GI.9 | HQ637267 |
GII/Hu/US/1971/GII.1/Hawaii | GII.1 | U07611 |
GII/Hu/GB/1994/GII.2/Melksham | GII.2 | X81879 |
GII/Hu/CA/1991/GII.3/Toronto 24 | GII.3 | U02030 |
GII/Hu/GB/1993/GII.4/Bristola | GII.4 | X76716 |
GII/Hu/GB/1990/GII.5/Hillingdon | GII.5 | AJ277607 |
GII/Hu/GB/1990/GII.6/Seacroft | GII.6 | AJ277620 |
GII/Hu/GB/1990/GII.7/Leeds | GII.7 | AJ277608 |
GII/Hu/NL/1998/GII.8/Amsterdam | GII.8 | AF195848 |
GII/Hu/US/1996/GII.9/VA97207 | GII.9 | AY038599 |
GII/Hu/DE/2000/GII.10/Erfurt546 | GII.10 | AF427118 |
GII/Po/JP/1997/GII.11/Sw918 | GII.11 | AB074893 |
GII/Hu/GB/1990/GII.12/Wortley | GII.12 | AJ277618 |
GII/Hu/US/1998/GII.13/Fayetteville | GII.13 | AY113106 |
GII/Hu/US/1999/GII.14/M7 | GII.14 | AY130761 |
GII/Hu/US/1999/GII.15/J23 | GII.15 | AY130762 |
GII/Hu/US/1999/GII.16/Tiffin | GII.16 | AY502010 |
GII/Hu/US/2002/GII.17/CS-E1 | GII.17 | AY502009 |
GII/Po/US/2003/GII.18/OH-QW101 | GII.18 | AY823304 |
GII/Po/US/2003/GII.19/OH-QW170 | GII.19 | AY823306 |
GII/Hu/DE/2002/GII.20/Luckenwalde591 | GII.20 | EU373815 |
GII/Hu/IR/2003/GII.21/IF1998 | GII.21 | AY675554 |
GII/Hu/JP/2003/GII.22/Yuri | GII.22 | AB083780 |
GIII/Bo/De/1980/GIII.1/Jena | GIII.1 | AJ011099 |
GIII/Bo/GB/1976/GIII.2/Newbury-2 | GIII.2 | AF097917 |
GIII/Ov/NZ/2007/GIII.3/Norsewood30 | GIII.3 | EU193658 |
GIV.1/Hu/NL/1998/GIV.1/Alphatron 98-2 | GIV.1 | AF195847 |
GIV.2/Fe/IT/2006/GIV.2/Pistoia 387 | GIV.2 | EF450827 |
GV/Mu/US/2002/GV.1/MNV-1 | GV.1 | AY228235 |
GV/Rn/HK/2011/GV.2/HKU_CT2 | GV.2 | JX486101 |
GVI/Ca/IT/2007/Bari 91 | GVI.1 | FJ875027 |
GVI/Ca/PT/2007/Viseu | GVI.2 | GQ443611 |
GVII/Ca/HK/2007/GVII/026F | GVII | FJ692500 |
Note: According to classification system of the online norovirus typing tool at http://www.rivm.nl/mpf/norovirus/typingtool (Kroneman et al., 2011)
The cryptogram is organized as follows: Genogroup/host species of origin /country of origin/year of occurrence/Capsid genotype/strain name
Host species abbreviations are: Bo, bovine; Ca, canine, Fe, feline; Hu, human; Mu, murine; Ov, ovine; Po, porcine; Rn, rat
Country abbreviations are: CA, Canada; DE, Germany; IR, Iraq; IT, Italy; JP, Japan; NL, Netherlands; NZ, New Zealand; PT, Portugal; SA, Saudi Arabia; UK, United Kingdom; US, United States
a The pandemic GII.4 variants and their GenBank accession numbers are: US95_96 (AJ004864), Farmington_Hills_2002 (AY485642), Asia_2003 (AB220921), Hunter_2004 (AY883096), Yerseke_2006a (EF126963), Den Haag_2006b (EF126965), NewOrleans_2009 (GU445325), and Sydney_2012 (JX459908) (Kroneman et al., 2013).
2.2.6. Genotyping System Based on the Viral RNA-Dependent RNA Polymerase
Table 3.5.3
Norovirus Genogroups and Genotypes as Determined by Polymerase (P) Gene Relatedness
Reference Virus | Genogroup. P Genotype | GenBank Accession Number |
GI/Hu/US/1968/GI.P1-GI.1/Norwalk | GI.P1 | M87661 |
GI/Hu/GB/1991/GI.P2-GI.2/Southampton | GI.P2 | L07418 |
GI/Hu/US/1998/GI.P3-GI.3/VA98115 | GI.P3 | AY038598 |
GI/Hu/JP/1987/GI.P4-GI.4/Chiba407 | GI.P4 | AB042808 |
GI/Hu/SE/2005/GI.P5-unknown/07_1 | GI.P5 | EU007765 |
GI/Hu/DE/1997/GI.P6-GI.6/BS5(Hesse) | GI.P6 | AF093797 |
GI/Hu/SE/2008/GI.P7-GI.7/Lilla Edet | GI.P7 | JN603251 |
GI/Hu/US/2008/GI.P8-GI.8/890321 | GI.P8 | GU299761 |
GI/Hu/FR/2004/GI.P9-GI.9/Chatellerault709 | GI.P9 | EF529737 |
GI/Hu/SA/1990/GI.Pa-GI.3/DesertShield | GI.Pa | U04469 |
GI/Hu/JP/2002/GI.Pb-GI.6/WUG1 | GI.Pb | AB081723 |
GI/Hu/JP/2000/GI.Pc-GI.5/SzUG1 | GI.Pc | AB039774 |
GI/Hu/FR/2003/GI.Pd-GI.3/Vesoul576 | GI.Pd | EF529738 |
GI/Hu/JP/1979/GI.Pf-GI.3/Otofuke | GI.Pf | AB187514 |
GII/Hu/US/1971/GII.P1-GII.1/Hawaii | GII.P1 | U07611 |
GII/Hu/GB/1994/GII.P2-GII.2/Melksham | GII.P2 | X81879 |
GII/Hu/CA/1991/GII.P3-GII.3/Toronto | GII.P3 | U02030 |
GII/Hu/GB/1993/GII.P4-GII.4/Bristol | GII.P4 | X76716 |
GII/Hu/HU/1999/GII.P5-GII.5/MOH | GII.P5 | AF397156 |
GII/Hu/JP/2002/GII.P6-GII.6/Saitama U16 | GII.P6 | AB039778 |
GII/Hu/JP/2002/GII.P7-GII.6/Saitama U4 | GII.P7 | AB039777 |
GII/Hu/JP/2002/GII.P8-GII.8/Saitama U25 | GII.P8 | AB039780 |
GII/Po/US/1997/GII.P11-GII.11/Sw918 | GII.P11 | AB074893 |
GII/Hu/JP/2005/GII.P12-GII.4/Sakai/04-179 | GII.P12 | AB220922 |
GII/Hu/FR/2004/GII.P13-GII.17/Briancon870 | GII.P13 | EF529741 |
GII/Hu/JP/2006/GII.P15-GII.15/Hiroshima66 | GII.P15 | AB360387 |
GII/Hu/DE/2000/GII.P16-GII.16/Neustrelitz260 | GII.P16 | AY772730 |
GII/Po/US/2003/GII.P18-GII.18/OH-QW101 | GII.P18 | AY823304 |
GII/Hu/DE/2005/GII.P20-GII.20/Leverkusen267 | GII.P20 | EU424333 |
GII/Hu/FR/2004/GII.P21-GII.2/Pont de Roide673 | GII.P21 | AY682549 |
GII/Hu/JP/2003/GII.P22-GII.5/Hokkaido133 | GII.P22 | AB212306 |
GII/Hu/JP/2004/GII.Pa-GII.3/SN2000JA | GII.Pa | AB190457 |
GII/Hu/US/1976/GII.Pc-GII.2/SnowMountain | GII.Pc | AY134748 |
GII/Hu/JP/2007/GII.Pe-GII.4/OC07138 | GII.Pe | AB434770 |
GII/Hu/FR/1999/GII.Pf-GII.5/S63 | GII.Pf | AY682550 |
GII/Hu/AU/1983/GII.Pg-GII.13/Goulburn Valley | GII.Pg | DQ379714 |
GII/Hu/JP/1997/GII.Ph-GII.2/OC97007 | GII.Ph | AB089882 |
GII/Hu/GR/1997/GII.Pj-GII.2/E3 | GII.Pj | AY682552 |
GII/Hu/JP/1996/GII.Pk-Unknown/OC96065 | GII.Pk | AF315813 |
GII/Hu/IN/2006/GII.Pm-GII.12/PunePC24 | GII.Pm | EU921353 |
GII/Hu/CN/2007/GII.Pn-GII.22/Beijing53931 | GII.Pn | GQ856469 |
Note: According to classification system of the online norovirus typing tool at http://www.rivm.nl/mpf/norovirus/typingtool (Kroneman et al., 2011)
Cryptogram is organized as follows: Genogroup/host species of origin/country of origin/year of occurrence/Pol (P) genotype-Capsid (C) genotype/strain name
Host species abbreviations: Hu, human; Po, porcine
Country abbreviations are: AU, Australia; CA, Canada; CN, China; DE, Germany; GB, Great Britain; GR, Greece; FR, France; HU, Hungary; IN, India; IT, Italy; JP, Japan; SA, Saudi Arabia; SE, Sweden; UK, United Kingdom; US, United States
Bold lettering represents P genotypes with matched C genotype numbering
2.2.7. Norovirus Online Genotyping Tool
3. Mechanisms for the generation of norovirus diversity
3.1. Genetic Drift

Evidence for two distinct mechanisms in the emergence of pandemic GII.4 noroviruses has been documented in molecular epidemiologic studies: antigenic drift and recombination (White, 2014). The VP1 capsid protein is organized into domains (Shell and Protruding) and subdomains (P1 and P2) (Prasad et al., 1999). A short N-terminal region (N) precedes the highly conserved S domain. The P2 domain, highly variable among strains, bears key antigenic and host carbohydrate ligand binding sites and is a major site of host selective immune pressure (Debbink et al., 2012). The asterisk represents a GII.4 P2 domain that has sustained amino acid substitutions that escape herd immunity. Proposed recombination breakpoints (BP) in GII.4 recombinants are indicated, with BPs identified at the ORF1/ORF2 junction, within ORF2, and near the ORF2/ORF3 junction (Eden et al., 2013). Examples of pandemic GII.4 variants and the mechanism by which they may have emerged are shown.
3.2. Transmission Bottlenecks
3.3. Recombination
4. Molecular epidemiology and transmission
4.1. Distribution of Norovirus Genotypes
4.2. Diversity and Norovirus Vaccine Development
4.3. Transmission and Site of Replication
5. Summary and future directions
Acknowledgments
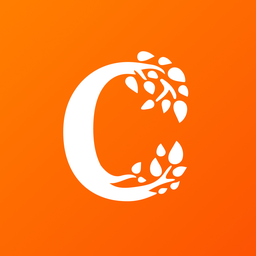
Full access? Get Clinical Tree
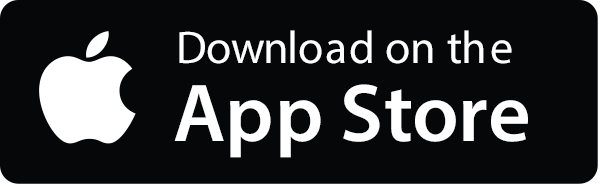
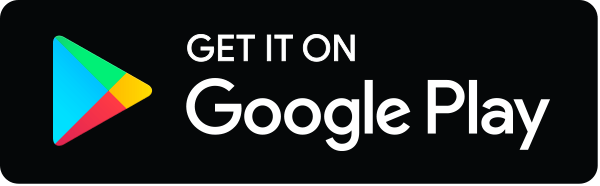