Fig. 11.1
Structural features of L. barbarum flavonoids
Moreover, rutin and kaempferol-3-O-rutinoside present absence of alkoxyl and glycoxyl ester groups on ring A. These facts explain the high antioxidant potency of them together with the fact that antioxidants act synergistically together with other antioxidants in vivo (Natella et al. 1999).
Another pathway of apparent antioxidant action of the flavonoids, particularly in oxidation systems using transition metal ions such as copper or iron, is chelation of the metal ions. Chelations of catalytic metal ions may prevent their involvement in Fenton-type reactions, which can generate highly reactive hydroxyl radicals.


The ability of polyphenols to react with metal ions may also render them prooxidants. The possible prooxidant effects of flavonoids may be important in vivo if free transition metal ions are involved in oxidation processes. In the healthy human body, metal ions appear largely sequestered in forms unable to catalyze free radical reactions. However, injury to tissues may release iron or copper, and catalytic metal ions have been measured in atherosclerotic lesions. In these cases the potential of flavonoids to act as prooxidants may increase (Croft 1998).
Phenolic acids may also be good antioxidants. They act as such in a number of ways. Phenolic hydroxyl groups are good hydrogen donors: hydrogen-donating antioxidants can react with reactive oxygen and reactive nitrogen species in a termination reaction, which breaks the cycle of new radical’s generation.
Following interaction with the initial reactive species, a radical form of the antioxidant is produced, having a much greater chemical stability than the initial radical. The interaction of the hydroxyl groups of phenolics with the π-electrons of the benzene ring gives the molecules special properties, most notably the ability to generate free radicals where the radical is stabilized by delocalization. The formation of these relatively long-lived radicals is able to modify radical-mediated oxidation processes (Pereira et al. 2009).
The naturally occurring phenolic acids share the frame structure of hydroxycinnamic acid or hydroxybenzoic acid and are present in many foods and plants. Research interests also arise for food quality since they are associated with color, sensory qualities, and nutritional properties. Recent interest has focused on their antioxidant properties and potential health. The antioxidant activity of phenolic acids is related to the number and position of hydroxyl groups in the molecule. The antioxidant efficiency of mono-phenols is strongly enhanced by the introduction of a second methoxyl or hydroxyl group at the o– or p-position with respect to the hydroxyl above (Castellano et al. 2012b; Szwajgier et al. 2005). According to previous reports, the introduction of a second hydroxyl group in the o-position (caffeic acid, chlorogenic acid, caffeoylquinic acid) or p-position (protocatechuic acid) enhances the antioxidant activity, making these phenolic acids more efficient than their respective monofenols (p-coumaric, syringic acid). This is consistent with the electron withdrawing potential of the single carboxyl functional group on the phenol ring affecting the o– and p-positions. Caffeic acid (hydroxycinnamic acid) is the most antioxidant compound. This is in agreement with the literature, in which hydroxycinnamic acids were found to be more effective than their hydroxybenzoic acid counterparts, possibly because of the aryloxy-radical stabilizing effect of the –CH = CH–COOH linked to the phenyl ring by resonance.
Moreover, we predict that the LB extract has an important antioxidant character, since it contains in addition to flavonoids, a great number of phenolic acid derivatives (benzoics and cinnamics), especially chlorogenic acid and p-coumaric acid, caffeic acid, and caffeoylquinic acid. However, in stress situations, the composition of phenols increases because these are formed to protect the plant from reactive oxygen species (ROS), anthropogenic pressures, and interspecific competition.
UV Absorption of the Main Flavonoids
Flavonoids as rutin, the main LB flavonoid, are synhetisized in higher plants to protect them from the harmful effects of UV-B radiation and diseases (Dong et al. 2009). The increased Ratio of Absorbance (MIRA) value of rutin under UV radiation indicated that rutin has the capacity of anti-UV and that cultivated LB leaves might be good sources for anti-radiation food or anti-UV cosmetics and protection to retinal diseases.
11.2.1.2 Antitumor Activity
Cancer is a multistep disease incorporating environmental, chemical, physical, metabolic, and genetic factors. It was found that in addition to their primary antioxidant activity, this group of compounds displays a wide variety of biological functions which are mainly related to modulation of carcinogenesis.
Natural phenolics can affect basic cell functions that are related to cancer development by many different mechanisms (Dai and Mumper 2010). They may limit the formation of the initiated cells by stimulating DNA repair (Webster et al. 1996).
Secondly, phenolics may inhibit the formation and growth of tumors by induction of cell cycle arrest and apoptosis (Dai and Mumper 2010). Polyphenols have been found to affect cancer cell growth by inducing apoptosis in many cell lines such as the hepatoma (HepG2), the colon (SW620, HT-29, CaCo-2, and HCT-116), the prostate (DU-145 and LNCaP), the lung (A549), the breast (MCF-7), the melanoma (SK-MEL-28 and SK-MEL-1), the neuroblastoma (SH-SY5Y), and the HL-60 leukemia cells.
Recently, many in vitro studies have been published on the modulation of oncogenes, tumor-suppressor genes, cell cycle, apoptosis, angiogenesis, and related signal transduction pathways by polyphenols (Yang et al. 2001).
11.2.1.3 Other Important Activities
There are some anti-inflammatory effects for LB flavonoids described, such as, for example, inhibition of the expression of intercellular adhesion molecule-1 (ICAM-1) and vascular cell adhesion molecule (VCAM-1) induced by tumor necrosis factor-α (TNF-α) in human umbilical vein endothelial cells (HUVECs) (Wu et al. 2012), and anti-atherosclerosis.
11.2.2 Beneficial Effects of Carotenoids from Goji Berries
The presence of two free carotenoids and seven carotenoid esters has been reported in wolfberries. Of the various carotenoids, the presence of zeaxanthin and its esters dominate the amount of carotenoids in wolfberries.
A high content of carotenoids provide high provitamin A value and antioxidant activity (Lin et al. 2011b). Zeaxanthin dipalmitate is the predominant carotenoid in Goji berries (Peng et al. 2005), followed by L-cryptoxanthin monopalmitate, zeaxanthin monopalmitate, L-carotene, and zeaxanthin (Inbaraja et al. 2008).
Zeaxanthin and lutein (isomeric dihydroxycarotenoids) are the major constituents of the retinal macular region (Bone and Landrum 1992). There are many studies showing the effect of Zeaxanthin in age-related macular degeneration (AMD) (see Sect. 11.4).
Zeaxanthin and other carotenoids are also potent antioxidants, which contribute to the health effects of Goji berries against the oxidative stress-mediated diseases (Zhong et al. 2013). Kim et al. studied the antihepatotoxic activity of zeaxanthin dipalmitate, showing a significant hepatoprotective activity against carbon tetrachloride toxicity and exerting a potent hepatoprotective activity by inhibiting Ito cell proliferation, collagen synthesis, and by inhibiting certain biochemical functions of Kupffer cells (Kim et al. 1997).
There are some studies about the effect of carotenoids such as zeaxanthin in cardiovascular diseases. For example, patients with coronary artery disease showed lower plasma levels of lutein, zeaxanthin, β-cryptoxanthin, α-carotene, β-carotene, and lycopene compared to healthy subjects. Moreover, the reduced levels of lutein, zeaxanthin, and β-cryptoxanthin were associated with smoking, high body mass index, and low high-density lipoprotein cholesterol (HDL-C) (Lidebjer et al. 2007). The antihypertensive effect of carotenoids is supported by a follow-up study in which the concentrations of the sum of four serum carotenoids (α-carotene, β-carotene, lutein/zeaxanthin, and cryptoxanthin) were inversely correlated with incident hypertension after 20 years (Hozawa et al. 2009).
Similar to other botanical (plant and fungal-derived) polysaccharides, LB polysaccharides (LBP) mainly occur as water-soluble glycoconjugates, for example, conjugates of glycan with peptides or proteins (Zhong et al. 2013).
In the last few years, the polysaccharides isolated from the aqueous extracts of LB fruits are described as one of the most valuable functional constituents and active compounds responsible for various health effects (Shan 2011).
Many studies on pharmacology and phytochemistry have demonstrated that LBP had various bioactivities such as antioxidant, immunomodulation, antitumor, antidiabetic, etc. (Jin et al 2013).
11.2.2.1 Antioxidant Activities
Many of the biological activities of LBP are directly or indirectly attributed to their antioxidant potential as many chronic diseases are oxidative stress-mediated (Zhong et al. 2013).
Antioxidants are substances that help reduce the severity of oxidative stress either by forming a less active radical or by quenching the reaction. It has been reported that LBP may possess the capacity to donate hydrogen to superoxide anion because of the weak dissociation energy of O–H bond (Jin et al. 2011).
Shan et al. analyzed the effects of LBP on exercise-induced oxidative stress in rats, showing that serum levels of MDA in an LBP-treated group were significantly decreased compared with that in the normal control group. Superoxide dismutase (SOD) and glutathione peroxidase (GPx) activities of rats (hind-limb skeletal muscle) in LBP-treated groups were significantly increased compared with that in the normal control (Shan et al. 2011).
In a similar study, Zhao et al. (2013) investigated the effects of LBP on arterial compliance during exhaustive exercise (swimming exercise) in rats. The rats administered LBPs showed longer swimming time until exhaustion than the control group rats. Exercise-induced MDA elevation was repressed by LBPs supplementation.
The LBPs significantly upregulated the expression of endothelial nitric oxide (NO) synthase (eNOS) and improved the endothelium-dependent vasodilatation of the aorta ring, showing that LBPs administration significantly inhibited the oxidative stress and improved the arterial compliance.
Along that same line, many authors described the effects of LBP in the decrease of MDA and beneficial increase of several antioxidant enzymes activities suggesting that the antioxidant activity of LBP is mainly attributed to the improvement of antioxidant enzymatic activities more than the donation of hydrogen to superoxide anion (Jin et al. 2011).
Some examples are the study of Amagase and Nance (2008) that investigated the antioxidant effects of LBP on 50 Chinese healthy adults aged 55–72 years in a 30-day randomized, double-blind, placebo-controlled clinical study. The results showed that LBP treatment significantly increased the serum levels of SOD by 8.4 % and GPx by 9.9 % and serum MDA decreased by 8.7 %, indicating that LBP could support health in humans by stimulating endogenous factors and protecting membranes from oxygen radical-mediated damage.
Recently, other antioxidant effects were studied (Chen et al. 2008). In this study, authors investigated the therapeutic effects of LBPs on learning and memory and neurogenesis in scopolamine (SCO)-treated rats. SCO administration led to damage of dendritic development of new neurons. LBP prevented these SCO-induced reductions in cell proliferation and neuroblast differentiation. LBPs decreased the SCO-induced oxidative stress in hippocampus and reversed the ratio Bax/Bcl-2 that was increased after SCO treatment. Those results suggest that suppression of oxidative stress and apoptosis may be involved in the above effects of LBPs that may be a promising candidate to restore memory functions and neurogenesis.
11.2.2.2 Antidiabetic Activities
Diabetes is associated with significant oxidative stress, and increasing evidences suggested that oxidative stress caused by hyperglycemia plays an important role in the pathogenesis of diabetes mellitus (Jin et al. 2012).
In 2004, Luo et al. studied the effects of purified LBP fractions in alloxan-induced diabetic or hyperlipidemic rabbits showing that those LBP fractions reduced blood glucose levels, total cholesterol (TC), and triglyceride (TG) concentrations at the same time that HDL levels markedly increased after 10 days of treatment in tested rabbits (Luo et al. 2004).
Along this line, Cui et al. (2011) and Wu et al. (2010) evaluated the effects of LBP on blood lipid metabolism, blood glucose, and oxidative stress of mice fed with high-fat diet. They found that LBP significantly decreased the levels of low-density lipoprotein (LDL), TC, TG, and blood glucose, and increased the activities of SOD, GPx, and catalase compared with high-fat diet groups.
11.2.2.3 Antitumor Effects
Some evidences have demonstrated that the anticancer activities of different chemotherapeutic agents are involved in the induction of apoptosis, which is regarded as the preferred way to manage cancer (Hsu et al. 2004). Zhang et al. (2005) investigated the effects of LBP on the proliferation rate, cell cycle distribution, and apoptosis in human hepatoma QGY7703 cell line. LBP treatment caused the inhibition of cell growth with cycle arrest in S phase and apoptosis induction. Mao et al. (2011) revealed that LBP treatment inhibited the growth of two different colon cancer cell lines (SW480 and Caco-2 cell lines) in a dose-dependent manner and cells were arrested at the G0/G1 phase.
The antitumor activity of LBPs seems to come from the induction of cell-cycle arrest and apoptosis, and inhibition of some signalling pathways, which play a protective effect against carcinogenesis by eliminating abnormal excess of tumor cells (Jin et al 2013).
11.3 Oxidative Mechanisms Involved in the Pathophysiology of Photoreceptor Cell Death in Retinal Diseases
The retina, located at the back of the eyecup, is a multilayer tissue with the main purpose of performing phototransduction: to transform photonic signals into electric energy that the brain is able to understand (Kolb 2003). Retinal cells can be altered in many ways and oxidative stress is known to play an important role in the development of several retinal diseases such as AMD, diabetic retinopathy (DR), and retinitis pigmentosa (RP) (Berson et al. 1993; Ganea and Harding 2006; Bazan 2006).
Even though the retina is part of the central nervous system and is also affected by oxidative stress (Halliwel 1992), it seems that photoreceptors are especially sensitive to oxidation due to its anatomical location and its function (Tanito et al. 2002). For that reason, survival of photoreceptors and other retinal cells depends on appropriate antioxidant mechanisms. Glutathione (GSH), together with different GSH-related enzymes, belongs to this antioxidant defense (Ganea and Harding 2006).
Retinitis Pigmentosa
RP is a group of heterogeneous inherited retinal degeneration that results in photoreceptor cell death (Hartong et al. 2006). Usually, rod photoreceptor cells are affected by a mutation and degeneration in certain time, whereas cone photoreceptors die secondarily even though they are not affected by the mutation (Sancho-Pelluz et al. 2008). RP produces nyctalopia or night blindness and reduction of peripheral vision (tunnel vision) which might develop to loss of central vision. Even though the primary cell death is, in most of the cases, well understood, secondary cell death of photoreceptors is still under study. Different mechanisms are discussed as causes of mutation-independent cell death of cones. Changes in oxygen consumption seem to be important in the rapid development of the disease. Loss of rods can be translated into a reduction of oxygen consumption and therefore a hyperoxia in the photoreceptor layer and an increase in oxidative stress in the remaining cells, both cones and surviving rods (Shen et al. 2005; Wellard et al. 2005). As seen earlier, ROS are normal products of mitochondrial metabolism. Protective mechanisms that include antioxidants and repair mechanisms have been confirmed to be active in the human retina (Puertas et al. 1993). An amplification of oxidative stress or a failure of the defense mechanisms leads to changes in metabolism which ultimately, if not resolved, may result in cell degeneration (Marcum et al. 2005). Oxidative stress has been implicated in the pathogenesis of RP. It was demonstrated that antioxidant therapies were able to slow down cell death rate in animal models of cone and rod degeneration (Komeima et al. 2006; Sanz et al. 2007). It is clear that redox status is imbalanced in a number of retinal maladies. In fact, in the majority of photoreceptor disorders, rod photoreceptor cell death is due to the genetic mutation, but cones, which are not affected by the mutation, must die in a mechanistically different way (Punzo et al. 2009). Lately, it has been demonstrated that mitochondria oxidative stress is an important source of ROS in different animal models of inherited photoreceptor degeneration (Vlachantoni et al. 2011).
The rd1 mouse, probably the best-known animal model for RP to date, holds a mutation in the gene that encodes for phosphodiesterase 6 (PDE6), producing a nonfunctional PDE6, essential for phototransduction (Bowes et al. 1990). Rod photoreceptor degeneration begins at postnatal day (P) 10, and is almost complete at P21; only cone cells remain at the photoreceptor layer. However, those cones will eventually die due to secondary nonmutation-related degeneration. An involvement of oxidative stress was found in this animal model for both mutation-induced rod cell death (Sanz et al. 2007) and secondary cone cell death (Komeima et al. 2006). Moreover, antioxidants were found to be protective in vitro studies of photoreceptor degeneration (Chucair et al. 2007).
Augmented oxidative stress may originate from either excessive energy demand, impairment of oxidative phosphorylation, or reduced antioxidant defenses. It has been hypothesized that Ca2 + concentration is elevated in the cytosol of rd1 rod cells. This might be caused because mutated PDE6 produces excessive accumulation of cyclic guanosine monophosphate (cGMP), and therefore the cGMP-activated cyclic nucleotide gated (CNG) ion channels are continuously open, leading to an Na + and a Ca2 + accumulation (Paquet-Durand et al. 2009). Elevation of intracellular Ca2 + produces the activation of mitochondrial calpain-10, which opens mitochondrial permeability transition pores (MPTP) (Arrington et al. 2006), which could explain translocation of apoptosis inducing factor (AIF) from mitochondria to the nucleus (Artus et al. 2006). Given that AIF has considerable redox activity, calpain-induced depletion of mitochondrial AIF would result in mitochondrial dysfunction and increased oxidative stress (Yamashima 2004).
It has been observed that the rd1 mouse also presents a decrease of defensive mechanisms against oxidative stress such as the action of GSH-S-transferase and GPx (Ahuja et al. 2008), where such downregulation is likely to enhance the deleterious effects of ROS.
11.3.1 Diabetic Retinopathy
It has been repeatedly suggested that oxidative stress is involved in the pathogenesis of late diabetes complications (Baynes and Thorpe 1996), though it is not definitely demonstrated if this is the cause or the consequence of these complications. It is clear that the elevated glucose levels present in diabetes and the existence of oxidative stress are inseparable (Packer et al 2001). The retina is extremely rich in polyunsaturated lipid membranes and this feature makes it especially sensitive to oxygen- and/or nitrogen-activated species and lipid peroxidation.
Hyperglycemia reduces antioxidant levels and concomitantly increases the production of free radicals; and in fact, in animal models of diabetes mellitus, reduced levels of retinal antioxidants, such as SOD, GSH reductase, and GPx, as well as nonenzymatic antioxidants such as vitamins C and E, and beta-carotene, have been observed. Levels of GSH, a key scavenger of ROS, are also reduced in the retina of diabetic rats (Muriach et al. 2006). Moreover, superoxide levels are elevated in the retina of diabetic rats and in retinal cells incubated in high-glucose media (Kowluru and Abbas 2003; Du et al. 2003; Cui et al. 2006), and hydrogen peroxide content is increased in the retina of diabetic rats (Ellis et al. 2000). Membrane lipid peroxidation and oxidative damage to DNA (indicated by 8-hydroxy-2-deoxyguanosine, 8-OHdG), the consequences of ROS-induced injury, are elevated in the retina in diabetes (Kowluru and Abbas 2003; Ellis et al 2000; Muriach et al. 2006; Miranda et al. 2004, 2006, 2007). These effects contribute to tissue damage in diabetes mellitus, leading to alterations in the redox potential of the cell with subsequent activation of redox-sensitive genes (Bonnefont-Rousselot 2002). Moreover, oxidative stress is linked to early apoptosis in DR both at the microvasculature and neuronal cells of the retina, but oxidative stress also appears to be highly interrelated with other biochemical imbalances that lead to structural and functional changes (Madsen-Bouterse and Kowluru 2008). Thus, for example, it is well known that chronic overproduction of ROS in the retina results in aberrant mitochondrial functions in diabetes (Kowluru 2005).
Among the proposed pathogenic mechanisms, the polyol pathway model has received the most scrutiny. Aldose reductase (AR) is the first enzyme in the polyol pathway, converting excess glucose to sorbitol, which is then metabolized to fructose by sorbitol dehydrogenase. According to several studies, AR is responsible for the early events in the pathogenesis of DR, leading to a cascade of retinal lesions including blood-retinal barrier (BRB) breakdown, loss of pericytes, neuroretinal apoptosis, glial reactivation, and neovascularization (Caldwell et al. 2005). Increased AR activity has been shown to contribute to increased oxidative stress by promoting nonenzymatic glycation and the activation of protein kinase C (PKC) (Stitt and Curtis 2005). It has been demonstrated that AR inhibition counteracts diabetes-induced oxidative and nitrosative stress and prevents vascular endothelial growth factor (VEGF) overexpression, basement membrane thickening, pericyte loss, and microaneurysms in retinal capillaries (Obrosova et al. 2003). In long-term diabetes-induced neuroretinal stress, increased expression of VEGF and apoptosis and proliferation of blood vessels have been shown to be less prominent than in AR-deficient animals (Obrosova et al. 2005).
A recent clinical study has substantiated the concept of hyperglycemic memory in the pathogenesis of DR. The Diabetes Control and Complications Trial-Epidemiology of Diabetes Interventions and Complications Research, has revealed that the reduction in the risk of progressive retinopathy resulting from intensive therapy in patients with type 1 diabetes persisted for at least several years after the Diabetes Control and Complications Trial (DCCT), despite increasing hyperglycemia. The process of formation and accumulation of advanced glycation end products (AGEs) and their mode of action are most compatible with the theoretical hyperglycemic memory (Yamagishi et al 2008). AGEs are formed by nonenzymatic reactions between reducing sugars and free amino groups of proteins or lipids. AGEs have been detected within retinal vasculature and neurosensory tissue of diabetic eyes. Multiple consequences of AGEs accumulation in the retina have been demonstrated, including upregulation of VEGF, upregulation of NF-κB, and increased leukocyte adhesion in retinal microvascular endothelial cells (Lu et al. 1998; Moore et al. 2003). In a 5-year study in diabetic dogs, administration of aminoguanidine (an inhibitor of AGEs formation) prevented retinopathy (Kern and Engerman 2001). AGEs exert cell-mediated effects via AGEs receptor (RAGE), a multiligand signal-transduction receptor of the immunoglobulin superfamily (Schmidt et al. 1992). Consequences of ligand-RAGE interaction include increased expression of VCAM-1, vascular hyperpermeability, enhanced thrombogenicity, induction of oxidant stress, and abnormal expression of eNOS (Schmidt et al. 1995; Wautier et al. 1996). Recently, it has been shown that after RAGE activation nicotinamide adenine dinucleotide phosphate (NADPH) oxidase is activated by phospholipase C-mediated activation of Ca2 + -dependent PKC and that this may lead to an increase in ROS that could be associated with the initial stages of macular edema and DR (Warboys et al. 2005). Studies in models of retinopathy show that increases in oxidative stress and signs of vascular inflammation are correlated with increases in arginase activity and arginase 1 expression; decreasing arginase expression or inhibiting its activity blocks these effects; and that the induction of arginase during retinopathy is blocked by inhibiting NADPH oxidase activity (Caldwell et al. 2010). Finally, it has been also demonstrated that AGEs can induce glial reaction and neuronal degeneration in retinal explants (Lecleire-Collet et al. 2005).
Alterations associated with oxidative stress offer many potential therapeutic targets making this an area of great interest to the development of safe and effective treatments for DR. Animal models of DR have shown beneficial effects of antioxidants on the development of retinopathy, but clinical trials (though very limited in numbers) have provided somewhat ambiguous results. Although antioxidants are being used for other chronic diseases, controlled clinical trials are warranted to investigate potential beneficial effects of antioxidants in the development of retinopathy in diabetic patients.
Age-Related Macular Degeneration
AMD is the major cause of blindness in the elderly with over 1.7 million people having reduced vision due to this disorder in the USA (Friedman et al. 2004). The disease affects the macula at the center of the eye and as a consequence results in loss of central vision which significantly impacts the patient’s ability to read, watch television, or drive. The major pathological changes associated with AMD are observed in the functionally and anatomically related tissues, including photoreceptors, retinal pigment epithelium (RPE), Bruch’s membrane, and choriocapillaries (Bhutto and Lutty 2012). AMD is broadly divided into two forms: dry and wet, that account for about 85 and 15 % of cases, respectively. Wet AMD, the most severe form of AMD, is generally associated with subretinal (i.e., between the retina and choroid) neovascularization and considerable amelioration can be achieved with the use of antiangiogenic agents (Andreoli and Miller 2007). Dry macular degeneration is mainly clinically diagnosed based on the presence of drusen, thickening of Bruch’s membrane and RPE progressive degeneration. Drusen are deposits of yellowish waste products from photoreceptors cells, which compromise their nutrition inducing ischemia and cell death, which in turn give rise to atrophy and finally results in irreversible visual loss (geographic atrophy). This disorder appears to consist of both a genetic and environmental component with a number of gene polymorphisms being identified which increase susceptibility to environmental risk factors such as smoking, hypertension, diet, or oxidative stress (Ding et al. 2009; Khandhadia and Lotery 2010; Montezuma et al. 2007; Swaroop et al. 2009; Ting et al. 2009). However, unequivocal proof of oxidative stress as a major causative factor in AMD, is difficult due to the complex nature of AMD and its restriction, in the true form of the disease, to humans (Beatty et al. 2001; Winkler et al. 1999; Zarbin 2004). In any case, there is considerable evidence to support oxidative stress as a contributing factor in the onset and progression of AMD as reviewed by Jarrett and Boulton (2012), with mitochondria representing a major source of endogenous ROS in the photoreceptors and underlying RPE (Jarrett et al. 2008; Liang and Godley 2003).
Recent studies show that pro-inflammatory cytokines, TNF-α, interleukin-1β, and interferon-γ, induce ROS in RPE cells via mitochondria and NADPH oxidase (Yang et al. 2007). The cross talk between NADPH oxidases and mitochondria may represent a “vicious cycle” of ROS production with mitochondria being a target for NADPH oxidase-generated ROS and mitochondrial ROS under certain conditions may stimulate NADPH oxidases. Moreover, studies have now begun to provide evidence supporting a role for polymorphic genes associated with oxidative stress at various stages of AMD (Jarrett and Boulton 2012), and recent studies have also implicated important roles for specific microRNAs in AMD. Thus, the miRNA, mir-23 is associated with increased RPE cellular resistance to oxidative stress and was found to be significantly downregulated in macular RPE isolated from AMD patients (Lin et al. 2011a). On the other hand, although light and oxygen are essential for vision they can also lead to photo-induced ROS and subsequent photochemical damage to the retina. The retina contains a variety of chromophores, which being excited at the appropriate wavelength can lead to significant photochemical damage, being the two major photosensitizers the visual pigments in photoreceptor cells and lipofuscin, which accumulates with age in the RPE (Boulton et al. 2001). Finally, other photoreactive molecules in the retina that can generate ROS under certain conditions include melanin, hemoglobin and other iron-containing proteins (e.g., cytochrome C), flavins, flavoproteins, and carotenoids (Boulton et al. 2001).
11.4 Evidences and Future Applications of Wolfberry Extracts in Retinal Diseases
Wolfberries extracts are widely used for the treatment of ocular disorders (Yang and Gao 2011). Most of the reports about the beneficial effects of LB compounds in different retinal diseases are studies about LB polysaccharides and carotenoids but not about polyphenol compounds.
Those research reports are mainly about LBPs and carotenoids effects on ischemia/reperfusion (I/R) injury (including glaucoma as a possible consequence), DR, and AMD. LBPs exert beneficial effects in animal models of ocular diseases. In fact, LBP extracts, are important water-soluble components which have protective effects in promoting survival and prolonging growth of retinal ganglion cells (RGCs) (Yang and Gao 2011) and they have been shown to protect RGCs in an animal model of chronic ocular hypertension (OH) (Chan et al. 2007).
In this part of the chapter, we review current and future applications of Goji berries in those retinal diseases.
11.4.1 Ischemia/Rerfusion Injury
There are many causes of retinal ischemia, including vein and artery occlusions, macular degeneration, diabetes, glaucoma, hypertension, etc. One of the complications after retinal I/R injuries is oxidative stress, which plays a role due to the high content of polyunsatured fatty acids in retina. ROS produced during I/R facilitate lipid peroxidation of membranes, denaturation of proteins, and DNA damage. Oxidative injury is accompanied by retinal swelling, neuronal cell death, and glial cell activation (He et al. 2014).
Many reports show the relationship between LBPs beneficial effects and retinal ischemia and the mechanisms of action proposed are many.
In this line, Li et al. studied the protective effects of LBPs against retinal I/R injury. They focus on three aspects apart from antioxidant activity: anti-apoptosis, preservation of BRB integrity and prevention of retinal swelling (Li et al. 2011). In this study, the authors treated mice with LBPs for 1 week prior to induction of ischemia and showed that LBP effectively protect the retina from neuronal death, apoptosis, glial cell activation, aquaporin water channel upregulation, disruption of BRB, and oxidative stress in retinal I/R induced by surgical occlusion of the internal carotid artery, 1 week after treatment with polysaccharides. Another study demonstrated that one possible mechanism for the protection of the retina by LBPs was immune modulation, which is an indirect effect; neuroprotective effects of LBPs were partly due to modulation of microglia activation (Chiu et al. 2009).
Cells have antioxidant defence systems to counteract oxidative stress. NF-E2-related factor 2/Antioxidant response element (Nrf2/ARE) pathway is one of the antioxidant pathways involved in counteracting increased oxidative stress and maintaining the redox status in many tissues (Ziaei et al. 2013; Kansanen et al. 2013).
One of the enzymes regulated by Nrf2/ARE is heme oxygenase (HO-1), which catalyzes the degradation of heme to biliverdin, CO, and iron. It is demonstrated that pharmacological induction of HO-1 protects the retina from acute glaucoma-induced ischemia-reperfusion injury (Sun et al. 2010).
He et al. studied the relation between the protection of LBPs against retinal damage induced by ischemia-reperfusion injury and activation of the Nrf2/HO-1 pathway. They saw that LBP pretreatment, not only reduced the generation of ROS, but also enhanced the activation of the Nrf2/HO-1 antioxidant pathway in I/R retinas. Furthermore, inhibition of HO-1 activity significantly blocked LBP-induced protective effects on I/R retinas, suggesting that the protective effects of LBP in I/R were mediated at least partly, by the activation of the Nrf2/HO-1 antioxidant pathway (He et al. 2014).
In this line, Chiu et al. suggest that LBPs have a neuroprotective effect on the survival of RGCs mediated via direct upregulation of neuronal survival signal βB2-crystallin. Crystallins are prominent proteins both in normal retina and in retinal diseases. These authors observed that rats fed with LBPs or with PBS did not change the crystallin profiles in the nonstress retinas. But the group, where chronic OH was induced and that was fed with LBPs, presented an increase of crystallin expression of more than tenfold, when compared with control (Chiu et al. 2010).
Mi et al. studied the protection of RGCs and retinal vasculature by LBPs in a chronic OH model (COH) of rat glaucoma. They observed a decrease in the expression of endothelin-1 (a potent vasoconstrictor synthesized in vascular endothelial cells) and its receptors (ETA and ETB) under COH condition (Mi et al. 2012a).
Acute OH (AOH) is another well-established animal model of retinal degeneration (Scarsella et al. 2012; Pescosolido et al. 1998). Mi et al. studied the protection of RGCs and retinal vasculature by LBPs in an AOH model suggesting a possible mechanism that consisted in downregulating the RAGE expression (when it is overexpressed in blood vessel, endothelial cells can produce the accumulation of amyloid-ß) and endothelin-1, as well as the related signalling pathways leading to amelioration of vascular damage and neuronal degeneration in AOH (Mi et al. 2012b).
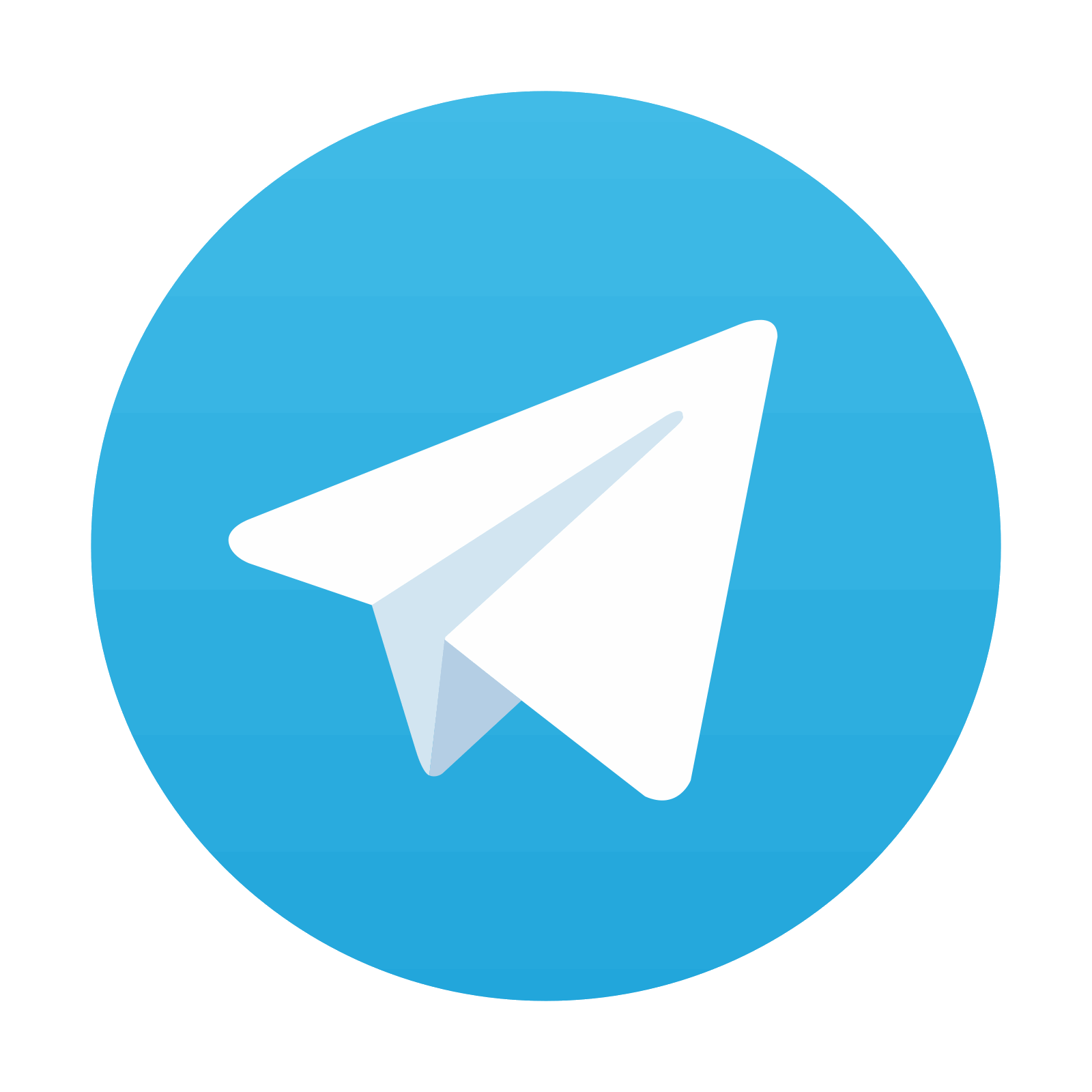
Stay updated, free articles. Join our Telegram channel
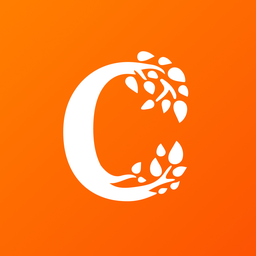
Full access? Get Clinical Tree
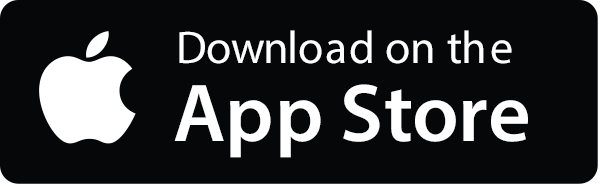
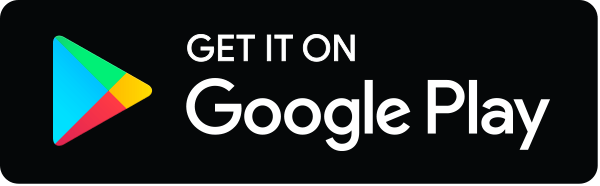