Fig. 5.1
Mechanism of RNA interference
5.5.1 Initiation
This stage is characterized by generation of siRNA mediated by type III endonuclease Dicer. It appears that the RNAi machinery, once it finds RNA duplexes, cuts it up into small molecules with a length of about 21 nt. These also have 2-nucleotide, 3′ overhangs, and 5′ phosphates. Of course, different organisms have different numbers of Dicer genes that process different sorts of dsRNAs. In Drosophila, Dicer-1 is seen to interact with the dsRBD protein Loquacious (Loqs). Immunoaffinity purification experiments have revealed that Loqs resides in a functional pre-miRNA processing complex, and stimulates and directs specific pre-miRNA processing activity. Dicer-2 requires R2D2, a dsRNA binding protein, which unlike Loq is supposed to be composed of two dsRNA binding domains. Both dsRNA binding domains of Dcr-2 and R2D2 are critical for Dcr-2/R2D2 complex to bind and load siRNA into siRISC complex.
In general, Dicer works by recognizing the ends of dsRNA with its PAZ domain (Dicer has PAZ domain at one end and dual RNase III domains on the opposing end) and then cutting the dsRNA with its RNase III domain. The distance between the PAZ and RNase III domain determines the length of siRNA produced by Dicer which varies by species. Long dsRNA and miRNA precursors are processed to siRNA/miRNA duplexes by the RNase-III-like enzyme Dicer. These short dsRNAs are subsequently unwound and assembled into effector complexes, RISCs, which can direct RNA cleavage, mediate translational repression or induce chromatin modification.
5.5.2 Effector
The second step of RNAi mechanism is referred as effector step; the incorporation of guide strand. This is characterized by the formation of silencing complex. The siRNA and miRNA duplexes that contain ribonucleoprotein particles (RNPs) are now made into RISC. Generally, effector complexes containing siRNAs are known as a RISC, while those containing miRNAs are known as miRNPs.
It is thought that RISC undergoes an ATP-dependent step that activates the unwinding of the double-stranded siRNAs. RISC is composed of PPD proteins (PAZ PIWI Domain proteins), which are highly conserved super-family. Members of PPD proteins contain PAZ (100 amino acids) and C-Terminal located PIWI (300 amino acids). The Argonaute protein and one strand of the siRNA form the RISC. Once the RISC complex has been formed from 5′-phosphorylated siRNAs and endogenous Argonaute protein, the siRNAs in the RISC complex guide the degradation that is sequence-specific, of the complementary or near complementary mRNAs. RISC binds to the mRNA which is targeted by the single RNA strand within the complex and cleaves the mRNA or represses their translation by homology-dependent m-RNA degradation. mRNA is cleaved in the middle of its complementary region. This cleaved mRNA cannot be translated. These newly synthesized siRNAs destroy the target with the help of RNA-dependent RNA polymerase (RdRP). Afterwards RISC dissociates and is ready to cleave other mRNAs. By that even a few numbers of the RISC can lead to high-level gene silencing. The siRNA derived in RNAi pathway may have two different fates, either it may be converted into holo-RISC for the destruction of the target m-RNA, or it may contribute to the amplification step by the generation of secondary siRNA.
RISC and miRNP complexes work by catalyzing hydrolysis of the phosphodiester linkage of the target RNA. The mechanism by which repression of translation guided by miRNA works, is not as well understood. It has been suggested that miRNAs affect translation termination or elongation rather than actual initiation of the process. Besides, miRNAs can act as siRNAs and vice versa. Perhaps mRNA degradation and translational regulation guided by miRNAs are used as simultaneous mechanisms for natural regulation.
5.6 Delivery Methods
RNAi has rapidly advanced from a laboratory observation into a major area of research within biology and medicine. The RNAi treatments are entering various stages of clinical trials. However, development of RNAi-based agents has been hindered because siRNAs are unstable in serum and delivery across the cell membrane is highly inefficient. Numerous methods have been developed to facilitate delivery of RNAi in animals and patients, each with their own set of advantages and disadvantages. To improve the effect of RNAi-based therapy, the enzymatic stability and cellular uptake of siRNA should be significantly enhanced, while their immunoactivation should be decreased. The two most common approaches for RNAi delivery are lipid mediated transfection and viral mediated transduction. Typically, researchers strive to achieve the highest levels of transfection efficiency possible. Transfection efficiency describes the percentage of cells that have received the RNAi duplex or expression plasmid. For many disease models, the most desirable cell types to use are primary cultures. However, these cannot be transfected adequately with commercially available transfection reagents. A powerful alternative to cationic lipid-mediated transfection is viral delivery of vectors expressing RNAi sequences. This option is the best for delivery to primary, hard-to-transfect, and non-dividing cells. Viral delivery can also be used to create stable cell lines with inducible RNAi expression or to express RNAi sequences with tissue-specific promoters.
5.6.1 Viral Methods
Since viruses work by delivering genetic material into a cell, scientists have been viewing them as a potential method of delivering DNA or RNA into a cell since long. Lentiviruses, a family of retroviruses that includes the human immunodeficiency virus (HIV), use RNA as their genetic material and have been used to deliver RNA to the cells of live mammals (including humans) for RNAi. Lentiviruses are valued among retroviruses for this purpose because they are the only ones that can work in non-dividing cells. Other viruses may also be used for RNAi delivery. Adenoviruses and herpes simplex-1 virus, both of which use double-stranded DNA instead of RNA, have been studied as RNAi vectors. Jonge et al. (2006) utilized virosomes derived from influenza as a means of delivering siRNA in vivo. Virosomes, which are empty influenza virus envelopes were complexed with cationic lipids for protection from nuclease activity and, following intraperitoneal injection, were demonstrated to successfully encapsulate siRNA duplexes and deliver them to cells in peritoneal cavity of mice. Virosomes may also serve as an effective means to target cells of the respiratory epithelium and cells that possess Fc receptors, such as dendritic-cells, macrophages, and natural-killer cells. Viral vectors have also been used to suppress the expression of HIV human co-receptor, chemokine receptor 5 to prevent HIV infection to penetrate lymphocytes. Such technologies are undergoing tests in a clinical trial (Nguyen et al. 2008).
Despite initial promise as a vehicle for the delivery of RNAi into live animals, viral vectors no longer appear to be the tool of choice due to adverse effects observed in viral vector gene therapy trials, and RNAi-induced hepatotoxicity.
5.6.2 Liposomal Delivery
For many immortalized cell lines, transfection with a lipid- or amine-based reagents is the preferred option. A major focus in the development of RNAi therapeutics is liposomal formulations for siRNA delivery to improve efficacy and safety. Many commercial institutions as reported by Nguyen et al. (2008) such as Silence Therapeutics plc are in the process of improving liposomal delivery. The work is in progress on a novel class of lipid delivery vehicles using ‘AtuPLEX,’ a mixture of cationic and fusogenic lipids complexed with negatively charged siRNAs, to promote uptake of siRNAs into endothelial cells of blood vessels in the liver and tumors.
Protiva Biotherapeutics Inc and Alnylam have utilized stable nucleic acid lipid particle (SNALP)-formulated siRNAs. SNALPs are specialized lipid nanoparticles that encapsulate siRNAs and are coated with a diffusible PEG-lipid conjugate which stabilizes the particle during formulation by providing a neutral hydrophilic exterior and prevents rapid systemic clearance in vivo by shielding the cationic bilayer; therefore, SNALPs facilitate the cellular uptake and endosomal release of the particle’s siRNA. In addition, Alnylam is exploring a library of ‘lipidoids’ or lipid-like molecules developed by Robert Langer and Daniel Anderson, to develop siRNA therapeutics for two different disease targets in the liver: hypercholesterolemia and liver cancer. Chemical modification and bioconjugation with lipids are known to improve the stability and cellular uptake of siRNA.
5.6.3 Chemical Modification
Chemical modifications to sugars, backbones, or bases of siRNAs have been shown to enhance their stability, prevent them from triggering an immune response, control their pharmacokinetic profiles and reduce nonspecific effects without affecting their biological activity. Most commonly used chemical modifications of siRNA include phosphorothiolation (P = S) of the non-bridging oxygen at the 3′-end, 2′-sugar modification (such as 2′-OMe or 2′-F) and locked nucleic acid (LNA). Chemical modifications of siRNA confer enhanced gene silencing at lower doses and reduced dosing frequency. In an experiment it was found that the placement of one, two or three ribonucleotides at the 5′ end of the modified siRNA of HBV263 and HBV1583 improved the median inhibitory concentration of the stabilized siRNAs by approximately fivefold.
5.6.4 Bioconjugation
A siRNA can be linked to a carrier molecule or covalently conjugated to a targeting ligand via a cleavable spacer to increase its cellular uptake or confer cell-specific targeting. To date, siRNA has been conjugated to lipids, polymers, peptides, and aptamers. Conjugation of siRNA to apatamer which can bind to prostate-specific membrane antigen (PSMA) has shown some promise for targeted delivery of siRNA to prostate cancer. siRNAs have also been conjugated to lipids like lithocholic and lauric acids at the 5′-end of the sense strand and showed increased cellular uptake of siRNAs in human liver cells without the use of any transfection reagent. Conjugated cholesterol to the 3′-end of the sense strand of siRNA targeting ApoB, an essential protein for assembly and secretion of very-low-density lipoprotein (VLDL) and low-density lipoprotein (LDL), has been formed that demonstrate significant increase in the levels of this conjugate in the liver.
5.6.5 Nanoparticle Delivery
The generation of nanosized particles is being investigated to enhance the delivery of siRNA based drugs and to circumvent the limitations of difficult to transfect cells such as lymphocytes. Numerous targeted nanoparticles have been used to overcome one or more of the barriers encountered by siRNA when trafficked to the cytosol. Antibody-protamine fusion bodies and antibody-targeted liposomes have been used successfully for primary lymphocytes to target human integrin lymphocyte function associated antigen-1 (LFA-1). Calando Pharmaceuticals Inc. has developed a cationic cyclodextrin-containing polymer that binds siRNAs for systemic delivery. Cyclodextrin containing polycation binds to the anionic backbone of the siRNA and then self-assemble into nanoparticles of approximately 50 nm in diameter.
5.6.6 Hydrodynamic Injection
Quick injection of siRNA in a large volume of physiological buffer effectively localizes duplex siRNA in the liver. In rats, administration of a VEGF (vascular endothelial growth factor)—specific siRNA resulted in more than 75 % inhibition of pathological neovascularization. Due to the invasiveness of the injection technique, hydrodynamics-based transfection is not appropriate for clinical applications at this point. However, recent advances in using a computer-controlled, catheter-guided injection device have greatly improved the precision and reproducibility of this approach. To date, the device has only been used for the delivery of DNA, but siRNA should be equally applicable in this approach.
5.6.7 Direct Administration into Target Organs
For dsRNA delivery several direct methods can be applied. Electroporation is used in simpler organisms, whereas microinjection of dsRNA into germ line or early embryo is preferred in multicellular organisms. In C. elegans, injection into the intestine or pseudocoelom is almost as efficient as injection into germ line. Even feeding worms with bacteria that express dsRNA or soaking worms in dsRNA solutions has been applied with some success. However, dsRNA injected into early embryos is diluted upon cell division; as a result early genes are more easily inactivated than late genes, which is especially a problem for higher organisms. In ongoing clinical trials of RNAi therapeutics, direct application of modified or unmodified siRNA into the eye (Allergen Inc. and Opko Health Inc.) and skin (TransDerm Inc.) through injection or by intranasal delivery (Alnylam) are the leading methods of administration. However, these delivery methods may be limited to a few indications in a small number of accessible organs such as the lung, eye and skin.
5.6.8 Delivery into Plants
siRNAs have been delivered into tobacco plants by biolistic pressure to cause silencing of GFP expression. Silencing occasionally was detected as early as a day after bombardment, and it continued to potentiate up to 3–4 days post-bombardment. Systemic spread of silencing occurred 2 weeks later to manifest in the vascular tissues of the non-bombarded leaves that were closest to the bombarded ones. After a month or so, the loss of GFP expression was seen in nonvascular tissues as well. RNA blot hybridization with systemic leaves indicated that the biolistically delivered siRNAs induced the de novo formation of siRNAs, which accumulated to cause systemic silencing.
5.7 Applications of RNAi
The new technique was taken up by lots of enthusiasm by scientists. It was soon realized that there has not been a tool this sharp in last many years. Besides being an area of intense, upfront basic research, the RNAi process holds the key to future technological applications.
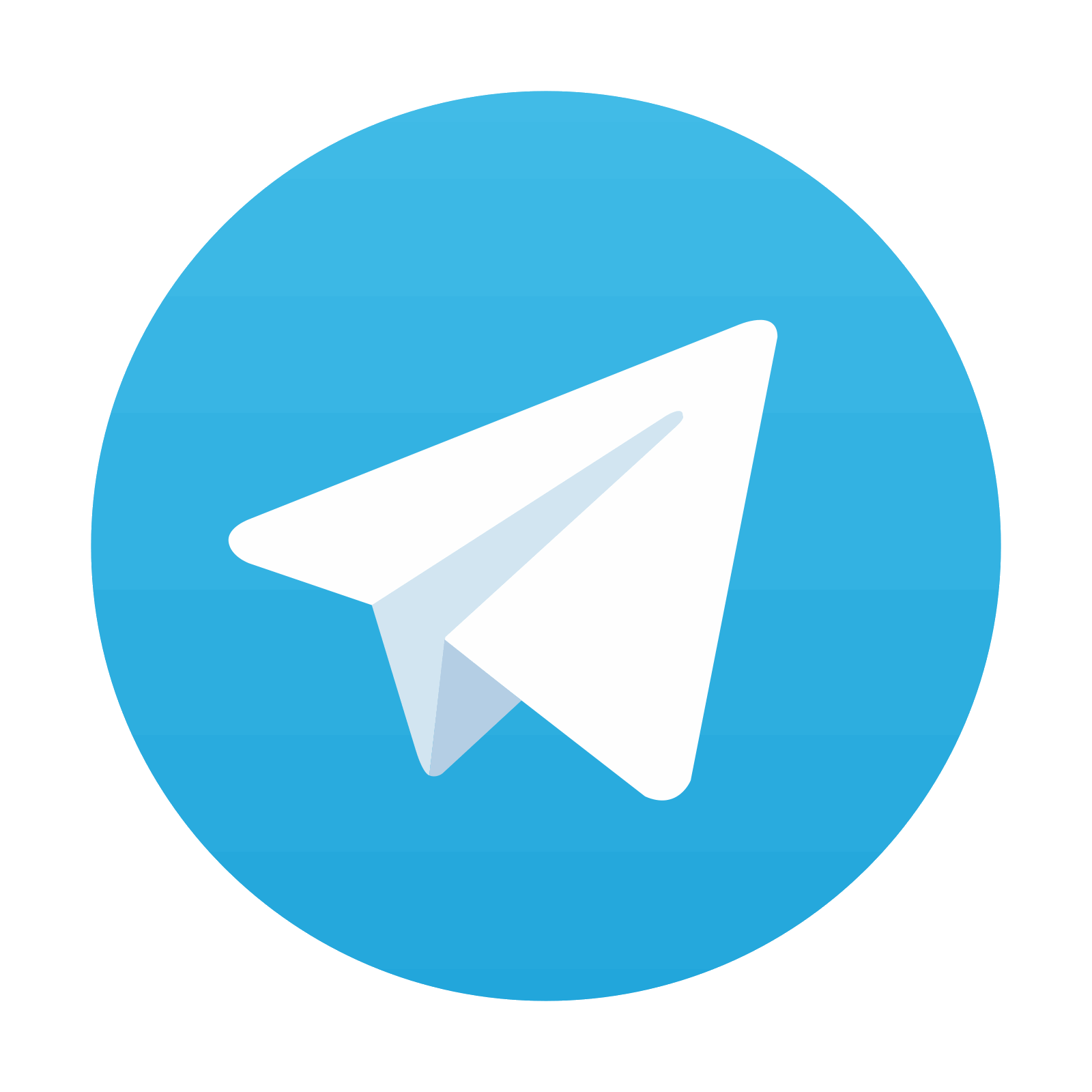
Stay updated, free articles. Join our Telegram channel
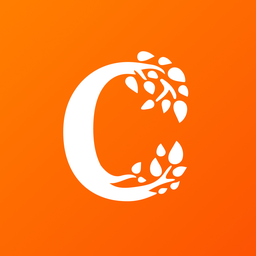
Full access? Get Clinical Tree
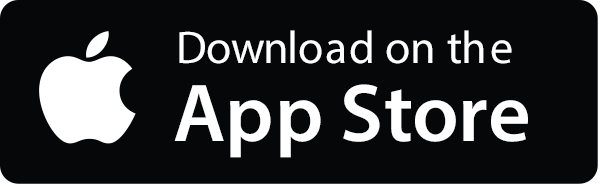
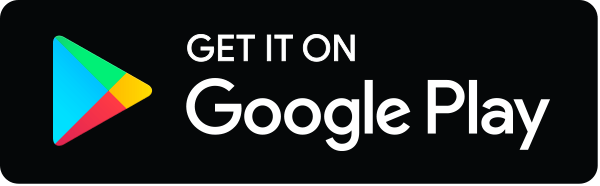