Riboflavin1
Hamid M. Said
A. Catharine Ross
1Abbreviations: EGRAC, erythrocyte glutathione reductase activity coefficient; FAD, flavin adenine dinucleotide; FMN, flavin mononucleotide; G6PD, glucose-6-phosphate dehydrogenase; Na+, sodium; RDA, recommended dietary allowance; RFT, riboflavin transporter.
Riboflavin (vitamin B2) was initially isolated from milk whey in a nonpure form in 1879 (1), followed by determination of its structure and identification of its major coenzymes, flavin mononucleotide (FMN) and flavin adenine dinucleotide (FAD) (2, 3, 4, 5). Although free riboflavin has little biologic activity, its coenzymes FMN and FAD play essential roles in normal cellular functions, growth, and development. Specifically, FMN and FAD act as cofactors for certain enzymes (flavoproteins) involved in electron transfer reactions (e.g., energy production reactions, metabolic conversion of essential micronutrients such as that of folate, vitamin B6, niacin), drug metabolism, toxin detoxifying, and electron scavenging pathways. Riboflavin deficiency, also called ariboflavinosis, leads to degenerative changes in the nervous system, endocrine dysfunction, anemia, and skin disorders as well as inflammation of the lining of the mouth, tongue, and throat; cracks at the corners of the mouth (angular cheilitis); and red and itchy eyes (from vascularization of the cornea). Riboflavin deficiency and suboptimal levels occur in persons with alcoholism and in patients with inflammatory bowel syndrome and diabetes, as well as in elderly persons.
CHEMISTRY, BIOCHEMISTRY, AND FUNCTION OF RIBOFLAVIN AND DERIVATIVES
The riboflavin molecule (7,8-dimethyl-10-(1′-D-ribityl)-isoalloxazine) is composed of an isoalloxazine planar ring to which a ribitol side chain is bound (Fig. 22.1). Free riboflavin has a molecular weight of 376.4, acts as a weak base in aqueous solutions, and is fluorescent. The vitamin has modest water solubility, which limits its use in parenteral and oral aqueous preparations. The riboflavin molecule is photosensitive and degrades to lumiflavin (7,8,10-trimethyl-isoalloxazine; Fig. 22.2) and lumichrome (7,8-dimethyl-alloxazine; see Fig. 22.2) at alkaline and neutral-acidic solutions, respectively. Both lumiflavin and lumichrome are biologically inactive compounds, yet they compete with riboflavin for uptake by different cells. Thus, prolonged phototherapy in neonates with jaundice and in patients with certain skin disorders may negatively affect normal body and cellular riboflavin homeostasis.
In the diet, riboflavin exists mostly in the form of FAD and FMN, with little in the free form. FMN is produced enzymatically by phosphorylation of the 5′-hydroxymethyl terminus of the ribityl side chain of the riboflavin molecule. This reaction is catalyzed by the enzyme flavokinase (Fig. 22.3). When FMN is further modified by the addition of a pyrophosphate-bridged adenyl moiety, a reaction that is catalyzed by FAD synthetase (also known as FAD pyrophosphorylase), the more abundant FAD form of the vitamin is produced (see Fig. 22.3). Conversion of free riboflavin to its coenzyme forms takes places mainly in the cytoplasm, although some conversion has also been reported to take place in the mitochondria (6, 7, 8, 9). Riboflavin conversion to FMN and FAD appears to be affected by thyroid hormones, an effect that appears to be mediated by flavokinase activation (10, 11, 12). Riboflavin can be regenerated from FMN and FAD in reactions that involve several phosphatases (see Fig. 22.3).
FAD is used more than FMN as a coenzyme by most cellular flavoproteins in the different metabolic pathways. In addition to generating a more biologically active form of the vitamin, cellular conversion of riboflavin to FMN and FAD also serves as a trapping and retaining mechanism for this essential micronutrient in the cell. Most of the intracellular flavoproteins are located in the mitochondria;
FAD (or riboflavin) is imported into this organelle by a mechanism that is different from that involved in the mitochondrial uptake of flavoproteins (9, 12, 13, 14, 15). Transport of FAD and riboflavin into the mitochondria appears to be occurred by a specific carrier-mediated system in the mitochondrial membrane (14). Little is known about how this system is regulated at the cellular and molecular levels and about the factors that affect its function.
FAD (or riboflavin) is imported into this organelle by a mechanism that is different from that involved in the mitochondrial uptake of flavoproteins (9, 12, 13, 14, 15). Transport of FAD and riboflavin into the mitochondria appears to be occurred by a specific carrier-mediated system in the mitochondrial membrane (14). Little is known about how this system is regulated at the cellular and molecular levels and about the factors that affect its function.
ASSESSMENT OF RIBOFLAVIN STATUS
Most flavins in the plasma exist in the form of free riboflavin, although some FMN and FAD are also present. All plasma flavins are associated with plasma proteins, and mostly with albumin. Two main methods are commonly used to assess riboflavin nutritional status. The first method is based on the determination of activity known as the erythrocyte glutathione reductase activity coefficient, abbreviated as EGRAC (16, 17), and the second is based on fluorometric measurement of urinary excretion of riboflavin over a period of 24 hours (expressed as total amount of riboflavin excreted or in relation to creatinine excretion). A newer method involving estimation of the activity of erythrocyte pyridoxine phosphate oxidase has been described (18). This method appears to be especially suited for use in populations with a high prevalence of glucose-6-phosphate dehydrogenase (G6PD) deficiency (18). Estimation of riboflavin status in patients with G6PD deficiency by means of the erythrocyte glutathione reductase activity method may mask riboflavin deficiency because G6PD deficiency is known to be associated with enhanced binding of FAD to erythrocyte glutathione reductase (19). Other methods available to measure the levels of riboflavin and its derivatives in biologic samples include high-performance liquid chromatography and binding of riboflavin and its derivative to specific flavoproteins (20).
PHYSIOLOGY OF RIBOFLAVIN
Intestinal Absorption
Humans and all other mammals cannot synthesize riboflavin, and thus they must obtain the vitamin from exogenous sources through intestinal absorption. The intestine therefore plays a central role in regulating and maintaining normal riboflavin body homeostasis. The intestine is exposed to two sources of riboflavin: a dietary source, which is processed and absorbed in the small intestine; and a bacterial source in which riboflavin is generated by the normal microflora in the large intestine and absorbed in that region of the gut (21). Riboflavin in the diet exists mainly in the form of FMN and FAD, which are noncovalently bound to proteins; free riboflavin exists only in small amounts in the diet. The first step in the processing of dietary FMN and FAD is their release from proteins by the combined action of gastric acid and intestine-associated hydrolases. The released FMN and FAD molecules are then hydrolyzed to free riboflavin in the intestinal lumen and surface through the action of alkaline phosphatases before absorption (22).
The mechanism of intestinal absorption of free riboflavin in the small intestine has been the subject of extensive investigations using various human and animal intestinal preparations. These preparations have ranged from intact intestinal tissue to purified vesicles isolated from the individual membrane domains of the polarized intestinal absorptive cells (i.e., from the apical brushborder membrane and from the basolateral membrane) (22, 23, 24, 25, 26, 27, 28, 29, 30). Collectively, these investigations have shown that absorption of free riboflavin occurs mainly in the proximal part of the small intestine and involves a specific sodium (Na+)-independent, carrier-mediated system. This system is inhibited in a competitive manner by riboflavin structural analogs, such as lumiflavin and lumichrome, and by amiloride (a Na+/hydrogen [H+] exchange inhibitor) (24). The intestinal riboflavin uptake process is also inhibited by chlorpromazine (a tricyclic phenothiazine drug), a compound that shares structural similarities with
riboflavin (25). Although some of the internalized riboflavin is phosphorylated inside the absorptive cells (31), only free riboflavin exits across the basolateral membrane. The latter process again involves a specific electroneutral, carrier-mediated mechanism (25).
riboflavin (25). Although some of the internalized riboflavin is phosphorylated inside the absorptive cells (31), only free riboflavin exits across the basolateral membrane. The latter process again involves a specific electroneutral, carrier-mediated mechanism (25).
The amount of riboflavin generated by the normal microflora of the large intestine depends on the type of the ingested diet. Higher amounts of riboflavin are produced following ingestion of a vegetable-based diet compared with a meat-based diet (32). In addition, considerable amounts of the bacterially produced riboflavin exist in the large intestinal lumen in the form of free riboflavin (32, 33), and thus are available for absorption. Indeed, studies have shown that the large intestine is capable of absorbing luminally introduced free riboflavin (34, 35). The mechanism involved in riboflavin uptake by colonocytes has been characterized and involves an efficient and specific carriermediated mechanism that is similar to the one described in the small intestine (36, 37). Considering the time that the luminal content resides in the large intestine, it is reasonable to assume that this source of riboflavin contributes to the host’s overall riboflavin nutrition, especially the cellular nutrition of the localized colonocytes. These colonocytes are known to depend on luminal content for other nutrients (e.g., short-chain fatty acids produced by bacteria are used by the colonocytes for energy production). Further studies, however, are needed to determine the exact level of contribution of this source of riboflavin to whole body riboflavin nutrition and the way in which environmental factors may affect such contribution.
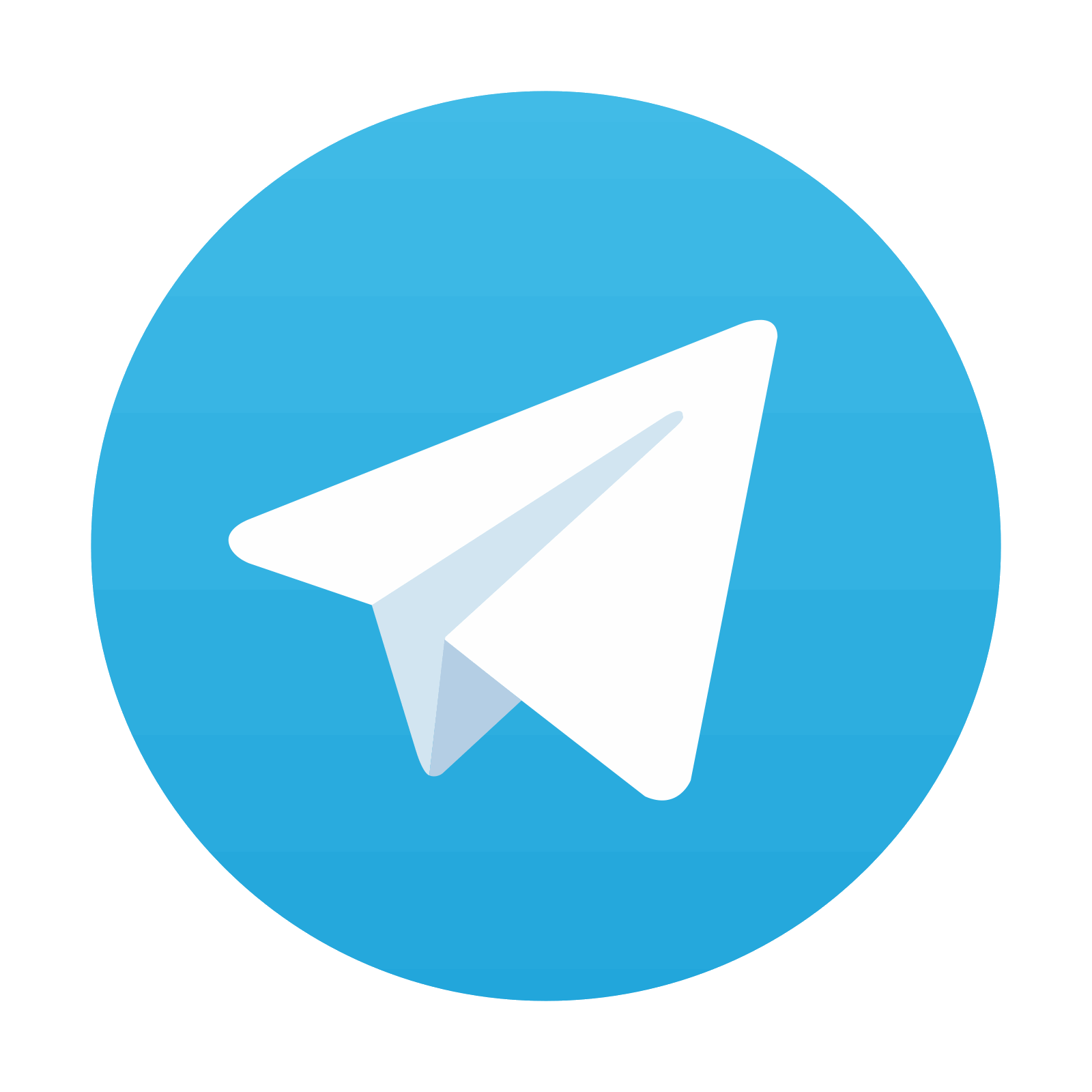
Stay updated, free articles. Join our Telegram channel
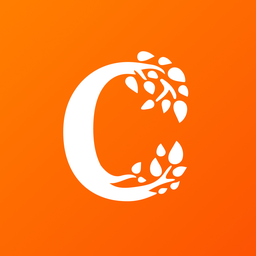
Full access? Get Clinical Tree
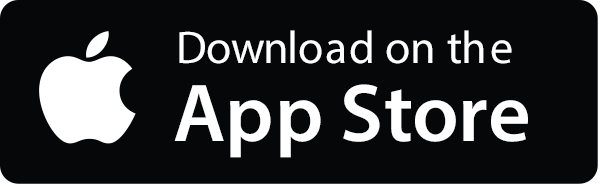
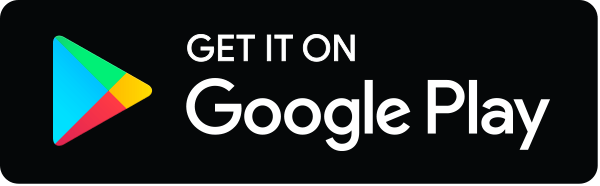