and Miguel A. R. B. Castanho2
(1)
Instituto de Bioquímica Médica Leopoldo de Meis, Federal University of Rio de Janeiro, Rio de Janeiro, Rio de Janeiro, Brazil
(2)
Institute of Biochemistry and Institute of Molecular Medicine, School of Medicine, University of Lisbon, Lisbon, Portugal
The survival of our ancestors was highly dependent on hunting, gathering, and fighting, behaviors that demanded intense physical activity. A sedentary lifestyle in that environment would certainly result in the elimination of the individuals. This situation imposed a selective pressure directed to adaptations of human physiology to a high capacity of physical activity, resulting in the development of a very efficient locomotor system, in which the skeletal muscles correspond to about 40 % of the body mass and account for a great proportion of the average energy consumption of the organism. The present-day sedentarism is dissonant with the human genetic background selected to favor a physically active lifestyle and probably consists in one of the main causes of the increasing incidence of modern chronic diseases, such as hypertension, obesity, and insulin resistance.
Muscles are tissues specialized in producing force and movement due to an amazing ability to convert the chemical energy of ATP phosphate bonds to mechanical work. This energy interconversion is performed by an array of proteins that forms a very organized structure inside the cells. The muscle mechanical activity may change very fast so that the energy sources and the metabolic pathway s used to maintain cellular functions need to be finely regulated.
In this chapter, we will discuss the metabolic adaptations to physical exercise, with special attention to the metabolism of the skeletal muscle cells. We will start with a brief review of the structure of the contractile apparatus and the mechanism of muscle contraction , followed by the description of the energy sources and the metabolic pathway s involved in muscle activity during physical exercise. We finish the chapter with the mechanism of action and the main metabolic effects of adrenaline, the major hormone secreted during exercise.
10.1 Muscle Contraction
Muscles are used either for locomotion or for the movements associated to the functions of the internal organs and are classified in three groups according to the type of movement they generate (Fig. 10.1). The skeletal muscles can be contracted voluntarily allowing the body to move and to maintain the posture, while rhythmic involuntary muscle contraction s , such as heart contraction or peristalsis and other autonomous motilities, are performed by cardiac and smooth muscles, respectively.
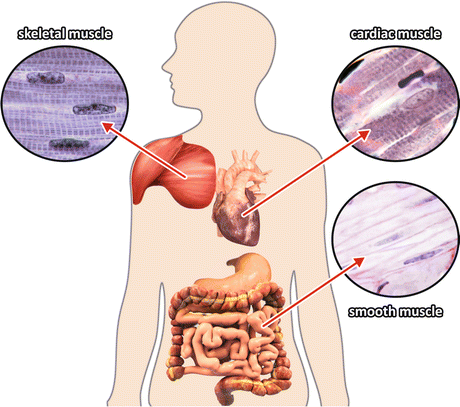
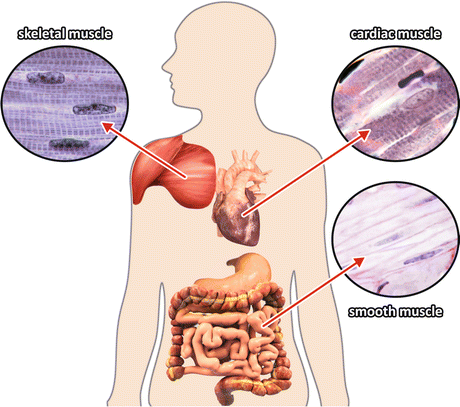
Fig. 10.1
The three types of muscle tissues. The details show histological images of skeletal, cardiac, and smooth muscles (Reprinted with the permission of Instituto de Histologia e Biologia do Desenvolvimento, Faculdade de Medicina, Universidade de Lisboa, FMUL)
10.1.1 Structural Organization of the Contractile Apparatus
In this chapter, we will focus our attention in skeletal muscles . This tissue is composed of parallel bundles of large multinucleated cells called muscle fibers. The fibers have 10–100 μm diameter and sometimes can extend over the full length of the muscle, reaching several centimeters. Most of the intracellular volume of the muscle fiber is occupied by 2 μm-thick myofibrils formed by the contractile array of proteins (Fig. 10.2a). Observed at the light microscope, the fiber presents a typical pattern that alternates light and dark bands caused by a regular arrangement of molecules of different densities (Fig. 10.2b).
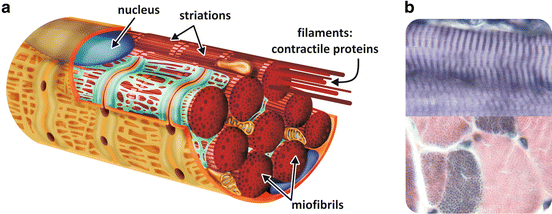
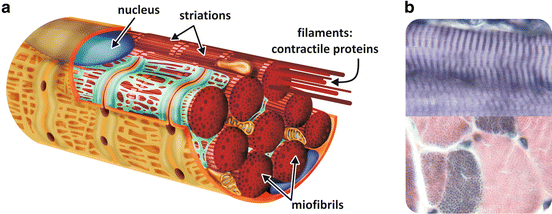
Fig. 10.2
(a) Schematic representation of a muscle fiber with the nucleus (blue) in the periphery of the cell and myofibrils formed by the contractile proteins . (b) Histological image of longitudinal (top) and transversal (bottom) sections of skeletal muscle tissue showing the striated pattern (Reprinted with the permission of Instituto de Histologia e Biologia do Desenvolvimento, Faculdade de Medicina, Universidade de Lisboa, FMUL)
The organization of the contractile proteins in the myofibril explains the striated pattern observed at the light microscope: they are arranged in an ordered structure forming thin and thick filaments, clearly seen by electron microscopy, as firstly observed by Hugh Huxley in the 1950s (Fig. 10.3). The different regions were named according to their characteristics. The dense regions are called A (from anisotropic) bands, while the less dense regions are named I (from isotropic) bands. A dark line in the medium point of each I band is also observed and is designated the as Z line (from the German word zwischenscheibe, which means “the disk in between”). A denser line is observed in the middle of the A band, called M line (from the German word mittelscheibe, which means “the disk in the middle”). In the resting muscle , the central region of the A band shows a lighter area, which is called H zone (from the German word heller, which means “brighter”). The I band is a region containing only thin filaments, while the A band contains both type of filaments. In the resting muscle, the thin filaments do not reach the center of the A band, explaining the H zone, which contains only thick filaments (Fig. 10.3).
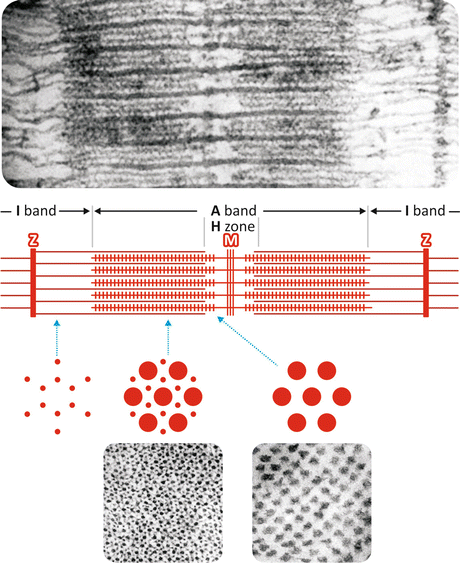
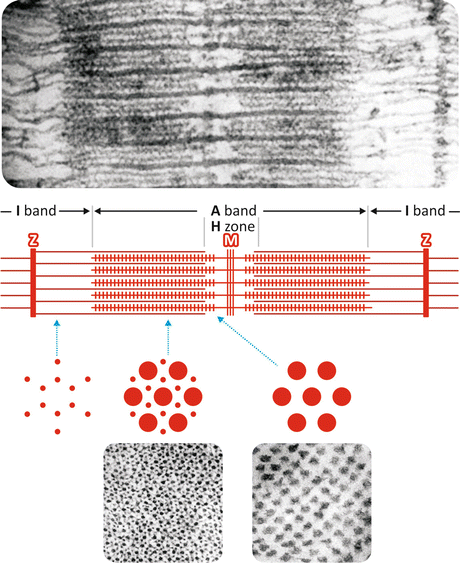
Fig. 10.3
Electron micrographs of a skeletal muscle (top) longitudinal section showing the thin and the thick filaments and (bottom) transverse sections of A band and H zone (Reproduced with permission from Huxley, J. Biophys. Biochem. Cytol. 3:631–648, 1957). The schematic representation shows filaments’ organization in the region in between two Z lines and transverse sections of the I band (left), the A band (medium), and the H zone (right). The region of 2–3 μm in between two Z lines is called sarcomere , which is the contractile unit of the myofibril
The striated pattern occurs in skeletal and cardiac muscles , which are called striated muscles, while the smooth muscles do not present striations.
The thin filaments are inserted in the Z line and are composed of three proteins : actin, tropomyosin, and troponin. The thick filaments are formed by a protein named myosin (see Box 10.1). The region comprised between the Z lines is called sarcomere and corresponds to the contractile unit of the myofibril . It is important to mention that the components of muscle cells are usually designated by a specific nomenclature having the prefix sarco (from a Greek root meaning “flesh”): the plasma membrane is known as sarcolemma, the cytoplasm is known as sarcoplasm, and the endoplasmic reticulum is called sarcoplasmic reticulum .
Box 10.1: Isolation of Myosin
Since the middle of the nineteenth century, it was known that the disruption of muscle cells resulted in the precipitation of an insoluble material in a much higher amount than that observed for homogenates of other tissues. Still in the nineteenth century, this insoluble material was called “myosin ” by Wilhelm Kuehne, but only in the 1940s, with the studies performed mainly by Albert Szent-Gyorgyi, this term was specifically used to name the proteic component that could be solubilized from the insoluble material by treatment with a high ionic strength solution (0.6 M KCl, an ionic strength much higher than the physiological 0.15 M). Another proteic component could be solubilized from the remaining insoluble material of muscle homogenate by lowering the ionic strength below the physiological range, and this protein was named “actin” (due to its ability to activate the ATPase activity of myosin, as it will be explained in the next section). It is important to note that both myosin and actin are insoluble at the physiological ionic strength, forming the organized filaments seen by electron microscopy.
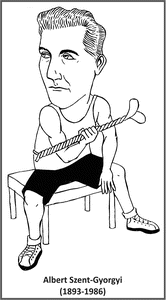
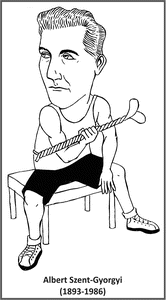
10.1.1.1 The Main Proteic Components of the Contractile Apparatus
Myosin represents up to 65 % of the total protein that constitute the myofibrils . A landmark finding regarding the role of myosin in contraction came from the work of W. A. Engelhardt and M. N. Lyubimova, who demonstrated that it displays enzymatic activity hydrolyzing ATP . It was also shown that the volume of myosin in its insoluble state (at ionic strength lower than 0.6 M; see Box 10.1) contracted after addition of ATP, leading to the supposition that the hydrolysis of ATP by myosin would be the driving force for muscle contraction . This is indeed the basis for the chemical–mechanical energy conversion during muscle contraction, as we will discuss in the next section.
The first electron microscopy images of isolated myosin filaments showed a very peculiar morphology. They present typical projections regularly spaced from each other along the extension of the filament, except for the central part, which is known as the “bare zone” (Fig. 10.4a). The projections are the contact points with the thin filaments.
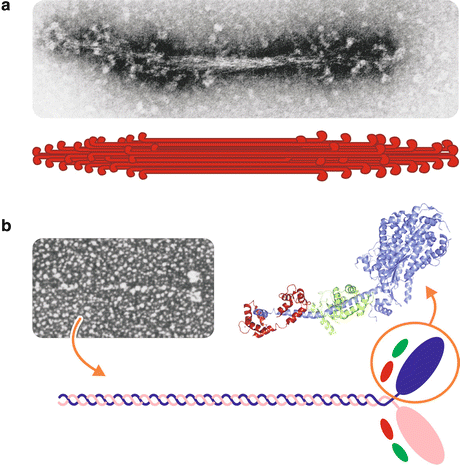
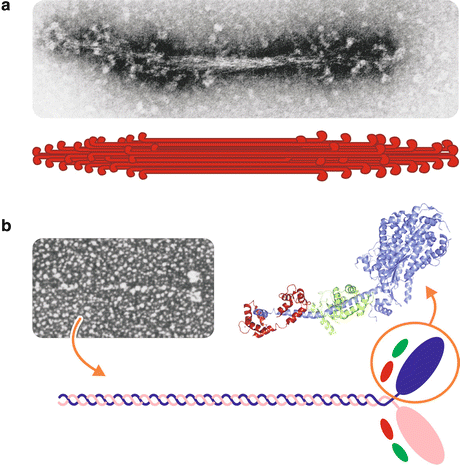
Fig. 10.4
Structure of the myosin filament. (a) Electron micrograph of a reconstituted myosin filament (Reproduced with permission from Huxley, Science 164:1356–1366, 1969) and its schematic representation. (b) Electron micrograph of an isolated myosin molecule (Reproduced from Slayter & Lowey, Proc. Natl. Acad. Sci. USA 58:1611–1618, 1967) and a schematic representation of its structure, which is composed of two heavy chains (purple and pink) associated to four light chains (green and red). The three-dimensional structure of the head, or S1 subfragment, is also shown, with the light chains highlighted in green and red (PDB 1DFL)
The morphology of the thick filament can be explained by the structure of myosin units. Each myosin unit in the thick filament shows a golf club shape, with two well-defined regions: a globular “head” from which a “tail” of about 150 nm long extends (Fig. 10.4b). It is a hexameric protein containing two identical heavy chains (with ~220 kDa each) and four light chains (each with ~20 kDa). The head can be separated from the whole myosin by brief digestion with proteases. The generated fragment, containing the N-terminal end of the heavy chains associated with the light chains, is called subfragment 1 (S1) and comprises the ATPase activity and the actin-binding site. The tail is composed of the C-terminal region of the two heavy chains intertwined to form a coiled coil.
Actin is the major component of the thin filament and corresponds to about 20–25 % of the muscle proteins . Actin is a protein of 42 kDa that is called G-actin (in a reference of its globular structure) in its monomeric form. To form the thin filaments, actin monomers polymerize in a helical structure, in which it is named F-actin (Fig. 10.5).
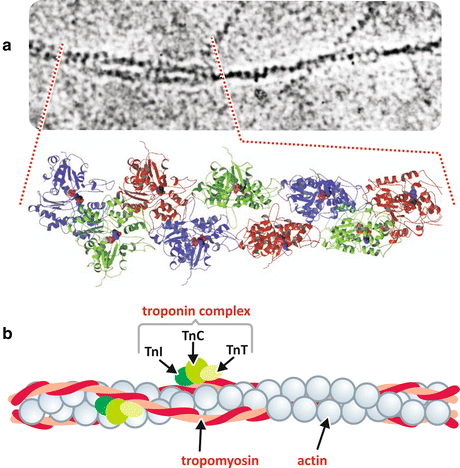
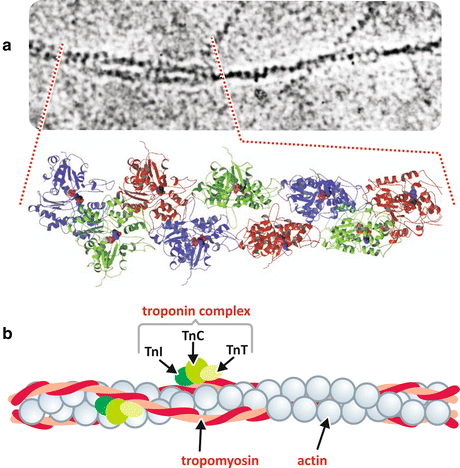
Fig. 10.5
Structure of the thin filament and its components. (a) Section of an electron micrograph from the article that firstly described the organization of an actin filament (Reproduced from Hanson & Lowy, J. Mol. Biol. 6:46–60, 1963, with permission from Elsevier), with the correspondent molecular structure determined by X-ray crystallography showing 13 actin monomers arranged on six left-handed turns repeated every 36 nm (Reproduced with permission from Geeves & Holmes, Ann. Rev. Biochem. 68:687–728, 1999). (b) Schematic representation of an actin filament with tropomyosin and troponin bound
Other proteins are associated to the thin filament. Among them, the most important are the tropomyosin and troponin, both involved in the regulation of contraction. Tropomyosin is a dimeric protein of 65 kDa that associates to actin as twisted α-helices that interacts in a tail-to-head manner forming long rods along the thin filament (Fig. 10.5b). Troponin is a globular protein of 78 kDa composed of three subunits, Tn-I, Tn-C, and Tn-T. Tn-I (I from inhibiting) binds actin fixing the tropomyosin-troponin complex on the actin surface and blocking myosin binding to thin filament. Tn-C (C from calcium binding) binds calcium ions (Ca2+), the main regulator of contraction (see Sect. 10.1.3). Tn-T (T from tropomyosin binding) promotes the association of the two other subunits of troponin and the binding of them to tropomyosin (Fig. 10.5b).
10.1.2 Mechanism of Muscle Contraction
After the discovery that the contractile apparatus was constituted mainly by two proteins , actin and myosin , many hypotheses have emerged to explain the contraction, most of them suggesting that muscle shortening during contraction was due to changes in protein structure leading to a more packed folding or coiling of the filament. However, the visualization of two separate sets of longitudinal filaments that overlap in certain regions in a series of studies performed during the 1950s led to a completely new model to explain the process.
10.1.2.1 The Sliding Filaments Model
The theory accepted today to explain myofibril shortening during muscle contraction was proposed in 1954, independently by H. E. Huxley and J. Hanson, and A. F. Huxley and R. M. Niedergierke, both studies published simultaneously. These scientists observed that either in contracted muscles or after their complete stretching, the length of the A band remained constant, while the length of the I band or the H zone varied according to the extent of contraction (Fig. 10.6). Based on these observations, they proposed that contraction occurred through the sliding of the filaments along each other.
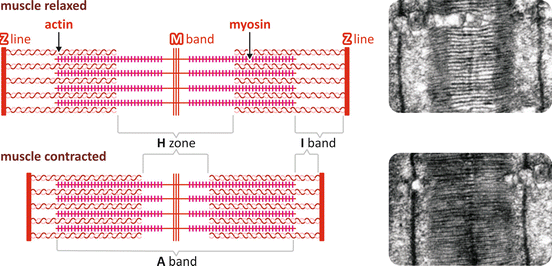
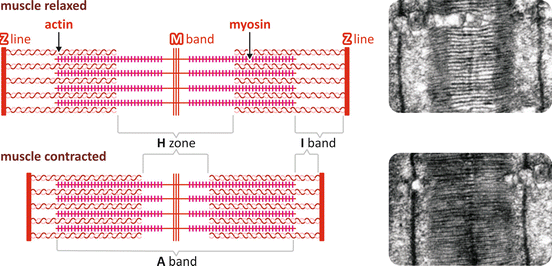
Fig. 10.6
Schematic representation of the slide of the filaments showing the relaxed and the contracted pattern with the corresponding electron micrographs (Reproduced with permission from Huxley. J. Biophys. Biochem. Cytol. 3:631–648, 1957)
One important finding that contributed to the elucidation of the mechanism that allows the filaments to slide was the observation that when the thin filaments were incubated with myosin S1, these subfragments form crossbridges with the thin filament in two orientations: perpendicular to the filament or with an inclination of approximately 45°. When inclined, all myosin heads show the same orientation on one side of the Z line but the opposite orientation on the other side of the Z line, which means that on each side of the Z line, the myosin heads point to opposite directions (Fig. 10.7a). This information could be correlated to the fact that during contraction the thin filaments on each side of the Z line are pulled to the center of the sarcomere .
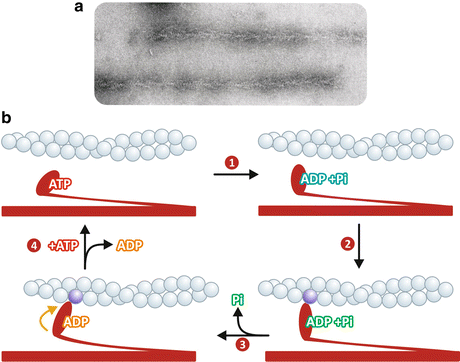
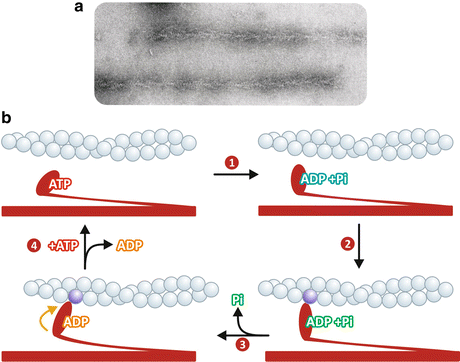
Fig. 10.7
(a) Actin filaments “decorated” with myosin heads, which show an arrowhead appearance (Reproduced with permission from Huxley, Science 164:1356–1366, 1969). (b) Schematic representation of actomyosin cycle: (1) myosin head hydrolyzes ATP to ADP and Pi ; (2) myosin heads bind to the actin molecule in a perpendicular orientation, in a pre-force-generating state; (3) Pi is released, leading to a conformational change that makes myosin head to be at a 45° orientation in relation to the filaments, allowing myosin to perform work; (4) ADP is replaced by ATP leading to myosin dissociation from actin
The current knowledge on the molecular mechanism that leads to the sliding of the filaments is based on the model proposed by R. W. Lymn and E. Taylor, known as the Lymn–Taylor actomyosin ATPase cycle, according to which contraction occurs as myosin heads bind and detach repeatedly to the thin filaments with concomitant ATP hydrolysis, each cycle with the binding occurring in a position closer to the Z line. The sequence of events that are repeated in each cycle can be summarized as follows, starting with ATP binding to the myosin head for convenience (Fig. 10.7b):
1.
Myosin hydrolyzes ATP to ADP and Pi , which remain tightly bound to the protein . The hydrolysis induces a structural change in the myosin head, which points to the thin filament in a perpendicular orientation.
2.
Myosin heads bind to the actin molecule in a pre-force-generating state.
3.
Binding of the myosin head to actin induces Pi to be released, leading to a conformational change that makes the myosin head to be at a 45° orientation in relation to the filaments. This conformational change allows myosin to perform work.
4.
ADP dissociates from myosin being replaced by ATP , whose binding makes myosin heads detach from the actin.
Thus, as the cycle is repeated, the thick filaments move along the thin filaments in the direction of the Z line, so that the H zone becomes shorter and the Z lines get nearer to each other.
It is important to note that if ATP is not available, myosin keeps strongly attached to the thin filaments, in a condition known as rigor, as occurs after death, when all the body muscles become rigid, which is called rigor mortis. In living muscle cells, on the other hand, there is always an excess of ATP due to a constant cycle of hydrolysis and resynthesis and thus myosin heads remain bound to actin only for short periods of time.
10.1.3 Regulation of Muscle Contraction
Knowing that ATP is constantly hydrolyzed and resynthesized within the cells, so that its levels remain almost unchanged, one would ask how muscle contraction can be triggered exactly when it is required and how it is stopped when a specific task has already been performed.
The answer resides in the fact that the process is controlled by the central nervous system . This means that although the contraction is sustained by ATP hydrolysis, the ATP levels inside the muscle cells do not regulate the process extensively. Conversely, ATP concentrations are maintained high by different metabolic pathway s that will be discussed in Sect. 10.2. Thus, it becomes clear that an additional player has to be called in to translate the brain signal to a biochemical response. This role is played by Ca2+, whose concentration transiently increases in the sarcoplasm inducing the start of the contraction.
To understand how Ca2+ concentration is modulated in the sarcoplasm to control contraction, we need firstly to look at the morphological organization of the muscle fibers. One particularity of the skeletal muscle cell is that its sarcolemma invaginates perpendicularly to the length of the cell forming what is called the transverse (T) tubules (Fig. 10.8). Inside the cell, the myofibrils are surrounded by a system of membranous vesicles called sarcoplasmic reticulum (SR ), which contains enlarged areas, known as the SR terminal cisternae, where Ca2+ is stored in a high concentration (~10−3 M). The SR terminal cisternae are connected to the T-tubules by a complex of two proteins , the dihydropyridine receptor (DHPR, inserted in the T-tubule membrane ) and the ryanodine receptor (RyR, inserted in the terminal cisternae membrane) (Fig. 10.8).
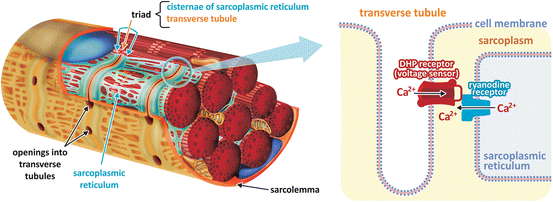
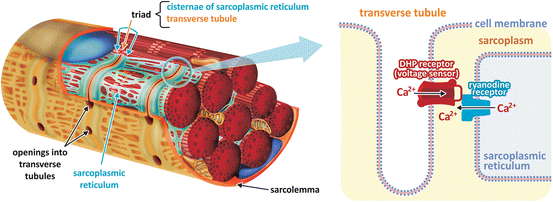
Fig. 10.8
Schematic diagram of a skeletal muscle fiber section showing the sarcolemma invaginations into the T-tubules and the sarcoplasmic reticulum with its terminal cisternae. Dihydropyridine receptor (DHPR), a voltage-dependent Ca2+ channel inserted in the T-tubule membrane , interacts with ryanodine receptor (RyR), also a Ca2+ channel , inserted in SR terminal cisternae membrane. Propagation of the action potential over the T-tubules activates DHPR, which in turn induces RyR opening, leading to Ca2+ release from SR lumen to the cytosol
A nerve impulse induces the opening of ion channel s in the sarcolemma leading to an inflow of Na+ into the cell. This causes membrane depolarization due to the dissipation of the membrane potential maintained by the Na+/K+-ATPase (see Box 10.2).
Box 10.2: Na+/K+-ATPase and the Maintenance of Cellular Membrane Potential
In living cells, the distribution of ions inside and outside the plasma membrane is asymmetric, resulting in an electrical voltage between the two sides of the membrane, which is called membrane potential. Membrane potential is regulated by the combined action of ion channel s , which depolarize the membrane, and ion pumps, which actively exchange ions across the membrane, restoring polarization. Membrane potential mainly arises from the exchange of Na+ and K+ through the activity of the Na+/K+-ATPase. This enzyme is an integral protein in the plasma membrane (see figure) that pumps 3 Na+ out in exchange of 2 K+ in, at the expense of ATP hydrolysis. Its activity accounts for a great part of cellular energy expenditure, being estimated that it is responsible for from 1/3 to 2/3 of ATP hydrolysis in the cells.
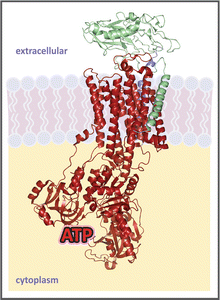
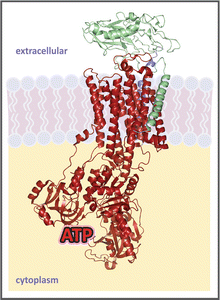
Crystal structure of Na+/K+-ATPase (PDB 3A3Y), with its three subunits, the α (catalytic, in red), β (in green), and regulatory (in blue). ATP is drawn in its binding site
Membrane depolarization propagates along the sarcolemma from the fiber surface to the T-tubules. DHPR is a voltage-dependent Ca2+ channel that is activated by the action potential propagation over the T-tubules. DHPR interaction with RyR in the closely apposed SR membrane causes RyR opening, leading to Ca2+ release from SR lumen to the cytosol. The increase of cytosolic Ca2+ concentration itself also activates RyR, causing further Ca2+ release, in a process known as Ca2+-induced Ca2+ release. At higher concentrations in the sarcoplasm, Ca2+ binds to troponin, resulting in a conformational change that induces the displacement of tropomyosin, allowing myosin heads to bind actin and starting the contraction cycle (Fig. 10.9).
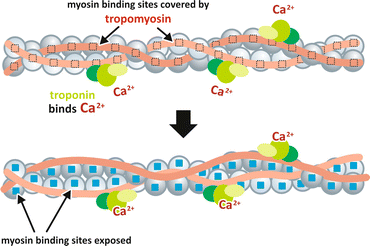
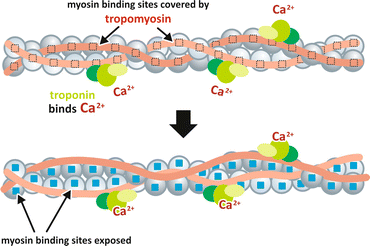
Fig. 10.9
Model for the Ca2+ regulation of contraction. At low concentration of Ca2+ in the sarcoplasm, the complex troponin–tropomyosin blocks the myosin -binding sites in actin. When Ca2+ is released from the SR and its concentration increases in the sarcoplasm, it binds troponin inducing a conformational change that ultimately leads tropomyosin to move away from the myosin-binding sites
When the nervous stimulus ceases, Ca2+ concentration in the sarcoplasm decreases due to the activity of Ca2+-ATPase, an SR membrane enzyme that pumps Ca2+ from the cytosol to the SR lumen. Thus, in resting muscle , the concentration of Ca2+ in the sarcoplasm is maintained very low, in the range of 10−7 to 10−8 M, since almost all the intracellular Ca2+ is stored inside the SR. In this situation, the complex troponin–tropomyosin is bound to actin in a way that prevents myosin binding (Fig. 10.9). Thus, even in high ATP concentrations, its hydrolysis by myosin occurs in a very slow rate and the muscle remains relaxed.
10.2 Different Metabolic Profiles of the Skeletal Muscle Fibers
Skeletal muscles are used to perform very different kinds of activities. Some of these activities require that muscle cells work in their maximal capacity, such as when an elite athlete runs a 100 m sprint, but also in more usual situations, as when you have to quickly run to take a bus that just started to leave the bus stop. Other activities demand muscle work for a long time, such as in running a marathon but also in prolonged walks, riding a bicycle, or cleaning the house. The different metabolic demands required in these diverse activities can be achieved due to the existence of distinct types of muscle fibers, characterized by specific metabolic adaptations that include the type of nutrient metabolized and the metabolic pathway s used to synthesize ATP (Fig. 10.10).
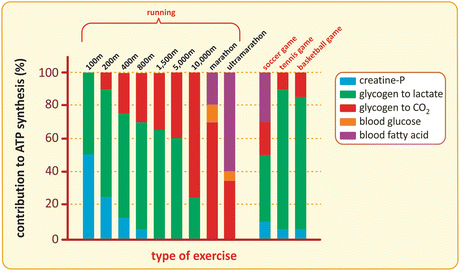
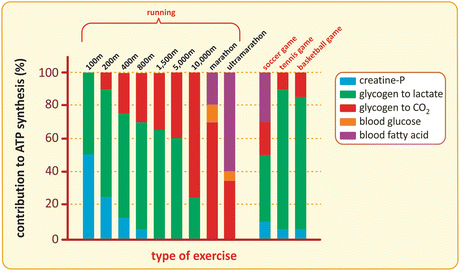
Fig. 10.10
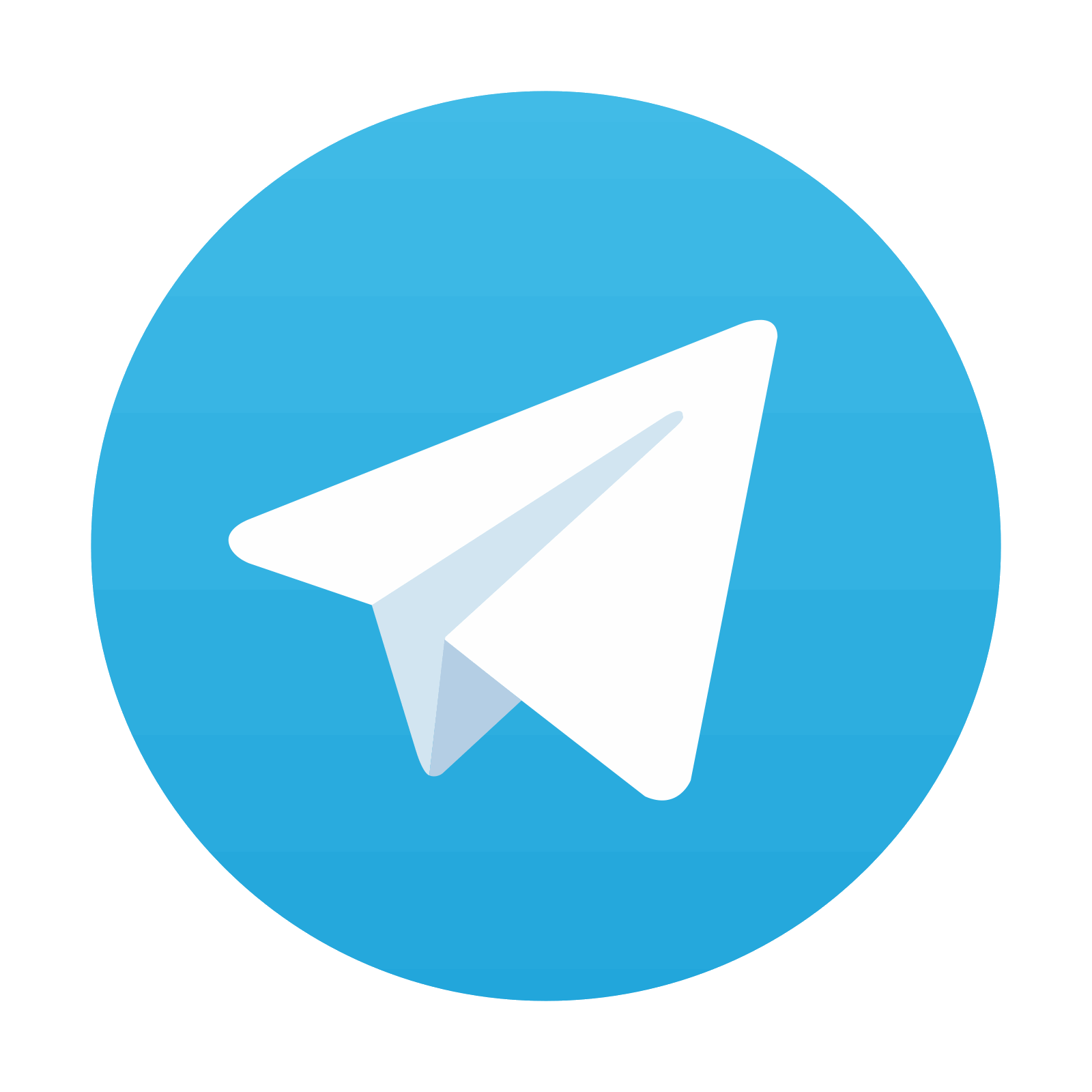
Contribution of distinct metabolites to ATP synthesis during different types of exercise. The transfer of the phosphate group of phosphocreatine to ADP is the fastest way to regenerate ATP in muscle cells (see next section for details), but the content of phosphocreatine is limited and sustains only short duration exercises. Muscle glycogen is the main energy source used for ATP synthesis during short- or medium-duration exercises. Depending on the type of exercise, muscle glycogen may be used anaerobically (for instance, in short-distance runs), generating lactate as the end product, or aerobically (as in long-distance runs), being oxidized to CO2 . Aerobic metabolism becomes gradually more important in long-duration exercises, with also an increasing requirement of fatty acid oxidation as glycogen is depleted. The use of blood glucose by muscle during exercise is almost irrelevant, especially because its transport into the muscle cells is dependent on insulin (see Sect. 8.4). This guarantees glucose availability for the cells that use this nutrient preferentially or exclusively, such as brain cells or erythrocytes , respectively. Ball games, such as soccer or tennis, may be long in duration but they consist in short and intense runs alternating with resting periods, which makes them having metabolite use profiles closer to short-distance running (Based on data from Newsholme & Leech, Functional biochemistry in health and disease, chap. 13. P. 291, 2010)
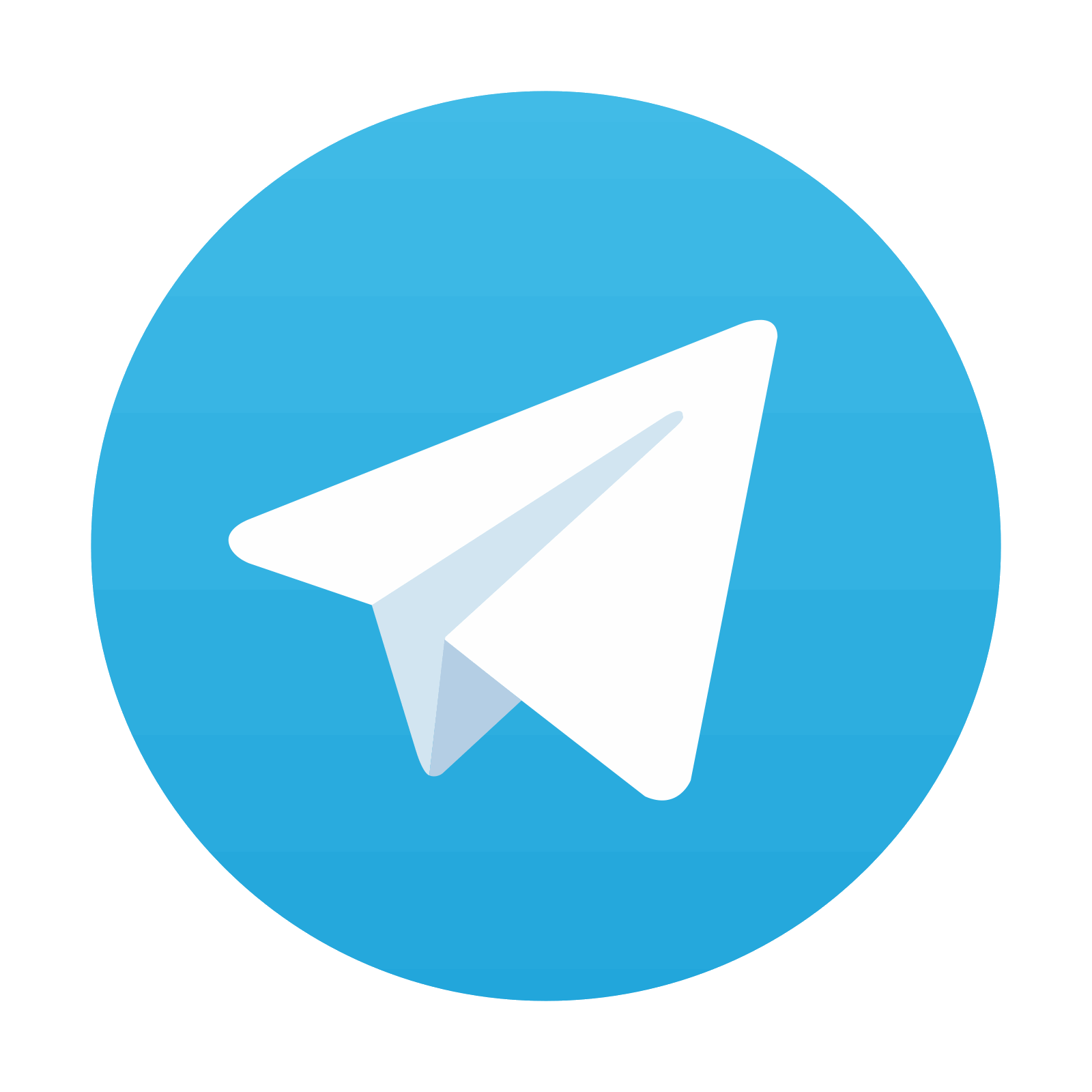
Stay updated, free articles. Join our Telegram channel
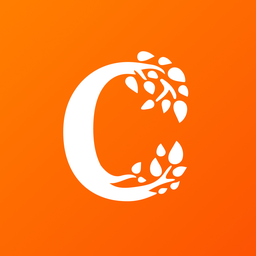
Full access? Get Clinical Tree
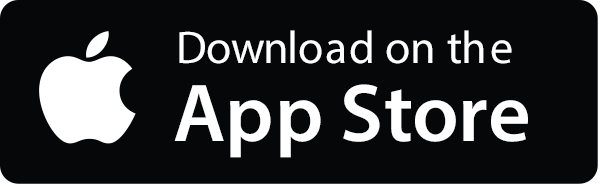
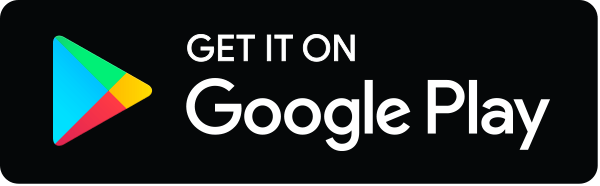
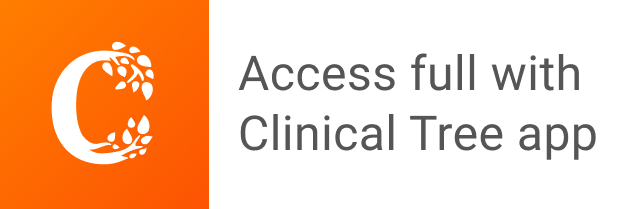