Box 36.1 USING ARM SPAN TO PREDICT A PATIENT’S STANDING HEIGHT
Arm span: height ratio
White males 1.019
White females 0.999
Black males 1.044
Black females 1.035
Predictive equation:
Height (cm) = 67.904868 + (Arm span) (0.664182) – (Sex*) (2.816175) – (Race*)(4.05492) – (Age in years) (0.070892)
NOTE: *For sex, male = 1 and female = 2; for race, white = 1 and black = 2.
SOURCE: Reprinted with permission of the American Thoracic Society. © American Thoracic Society. From Parker JM, Dillard TA, Phillips YY. Arm span-height relationships in patients referred for spirometry. Am J Respir Crit Care Med. 1996;154:533–536.
The percent predicted values for FEV1 and FVC can also be used to grade disease severity. Deficits are typically described as mild, moderate, moderately severe, severe, or very severe depending on the degree of deviation from the predicted values (see table 36.1).
Table 36.1 USING CONFIDENCE INTERVALS TO GRADE SEVERITY OF DEFICITS IN SPIROMETRY
For Both Fev1 and FVC (Using Confidence Intervals) | |
Normal | <1.0 confidence interval from predicted value |
Mild deficit | ≥1.0–1.75 confidence intervals from predicted value |
Moderate deficit | ≥1.75–2.50 confidence intervals from predicted value |
Severe deficit | ≥2.50 confidence intervals from predicted value |
For FEV1 (Using Percentage Predicted) | |
Mild | >70% predicted |
Moderate | 60–69% predicted |
Moderately severe | 50–59% predicted |
Severe | 35–49% predicted |
Very severe | <35% predicted |
In addition to being characterized numerically, the measurements obtained while testing spirometry (FVC, PEFR) are typically displayed as a flow-volume loop. The flow-volume loop displays the expiratory effort with volume (in liters) on the x axis and flow (in liters per second) on the y axis. The expiratory effort starts at the intersection of the axes and terminates when the loop again intersects the x axis (i.e., when flow again equals zero). The flow-volume loop typically displays the immediate posttest inspiration (below the x axis, when flow is negative). The shape of the flow-volume loop can be helpful in assessing the expiratory effort and the presence of certain disease states (see Lung Volumes: Measurements and Values below).
A less-than-maximal expiratory effort is present when the terminal aspect of the inspiratory portion of the flow-volume loop (the portion below the x axis, when flow is negative) intersects the x axis left of the y axis. This implies that the subject did not maximally inhale prior to the expiratory effort or there was an air leak during exhalation (see Figure 36.1). Other examples of less-than-maximal effort include a blunted peak flow or an abrupt drop to zero flow.
Figure 36.1. Flow-volume loop in a normal individual (A) and in an individual demonstrating submaximal expiratory effort (B). Source: Reprinted with permission from Miller MR, Hankinson J, Brusasco B, et al. Standardisation of spirometry. Eur Respir J. 2005;26:319–338. © 2005 European Respiratory Society.
A classic finding of obstructive ventilatory disease is a “coved” appearance of the flow-volume loop. As discussed below (see Obstructive Ventilatory Deficits), airway resistance is increased in obstructive ventilatory deficits due to narrowing of the airway lumen (i.e., bronchospasm in asthma or airway collapse in emphysema). The coved appearance of the flow-volume loop reflects this increased airway resistance as velocity of flow decreases precipitously compared to predicted values (see Figure 36.2). Airways resistance is decreased in patients with restrictive ventilatory deficits, while lung volumes are reduced overall (see Restrictive Ventilatory Deficits below), and the flow-volume loop may reflect this. PEFR may be higher than expected even though the FEV1 and FVC may be reduced, and the FEV1/FVC ratio may be preserved or even elevated (see Figure 36.3). Finally, a fixed upper or central airway obstruction may cause a limitation on the upper maximum of flow velocity; the flow-volume loop reveals a decrease in PEFR with a “plateau” appearance, without reduction in overall FVC (see Figure 36.4). FEV1 and FVC are not usually decreased in upper or central airway obstruction.
Source: Reprinted with permission from Miller MR, Hankinson J, Brusasco B, et al. Standardisation of spirometry. Eur Respir J. 2005;26:319–338. © 2005 European Respiratory Society.
Figure 36.2. Flow-volume loop in individuals with obstructive ventilatory deficits: asthma (A) and COPD (B).
Figure 36.3. Flow-volume loop an individual with a restrictive ventilatory deficit. Source: Reprinted with permission from Pellegrino R, Viegi G, Brusasco B, et al. Interpretative strategies for lung function tests. Eur Respir J. 2005;26:948–968. © 2005 European Respiratory Society.
Figure 36.4. Flow-volume loop in fixed upper airway obstruction. Source: Reprinted with permission from Miller MR, Hankinson J, Brusasco B, et al. Standardisation of spirometry. Eur Respir J. 2005;26:319–338. © 2005 European Respiratory Society.
LUNG VOLUMES: MEASUREMENT AND VALUES
The lung volumes of most clinical interest include the total lung capacity (TLC—the volume of air in the lungs after maximal inhalation), residual volume (RV—the volume of air remaining in the lungs after maximal exhalation), and functional residual capacity (FRC—the volume of air in the lung after a normal tidal volume breath is exhaled). The various lung volumes and lung capacities are depicted in Figure 36.5. In brief, lung volumes are the RV, expiratory reserve volume (ERV), tidal volume (VT), and inspiratory reserve volume (IRV). Lung capacities are the sum of two or more lung volumes. The lung capacities are the inspiratory reserve capacity (IRC = VT + IRV), vital capacity (VC = ERV + IRC), functional residual capacity (FRC = RV + ERV), and TLC (RV + ERV + VT + IRV or VC + RV or FRC + IRC).
Figure 36.5. Lung volumes and capacities. Source: Reprinted with permission from Wanger J, Clausen JL, Coates A, et al. Standardisation of the measurement of lung volumes. Eur Respir J. 2005;26:511–522. © 2005 European Respiratory Society.
Lung volumes are typically measured by one of three techniques: helium dilution, nitrogen washout, or body plethysmography.
The nitrogen washout method determines lung volumes by comparing the initial concentration of nitrogen in the alveoli and the concentration after breathing 100% oxygen for up to 7 minutes (to “wash out” alveolar nitrogen). Although truncated protocols have been proposed (i.e., breathing 100% oxygen for 5 as opposed to 7 minutes), the nitrogen washout method of measuring lung volumes is time consuming compared to plethysmography.
Plethysmography is based on Boyle’s law: P1V1 = k, where k is a fixed constant. Technically, lung volumes are measured by plethysmography in a sealed chamber in which the volume of air is known and constant (referred to as a variable-pressure chamber). Because people enter into and are sealed within this chamber during testing, the chamber is colloquially referred to as a “body box.” There is a mouthpiece within the chamber through which the subject breathes. When the respiratory cycle is at or near FRC, the mouthpiece is occluded by an automated shutter valve. The subject then pants (attempting to inhale and exhale against the closed mouthpiece). The change in pressure at the mouthpiece, the change in pressure in the plethysmograph, and the known fixed volume of gas in the plethysmograph can be used to calculate the quantity of air in the lungs at FRC. After determining the volume of air in the lungs at FRC, exhalation to RV followed by inhalation to TLC is performed to determine the values of ERV and inspiratory vital capacity (IVC), respectively. Plethysmography may overestimate lung volumes. The most important example of this overestimation of lung volumes occurs in subjects with obstructive airways deficits and “air trapping” (see Obstructive Ventilatory Deficits below for definitions of air trapping and hyperinflation). When the shutter valve on the mouthpiece is closed and the subject pants, the assumption is that there is no flow of air (given the closed mouthpiece) and that changes in pressure reflect only changes in volume of the air in the lungs. However, particularly in patients with obstructive deficits, there may be flow between alveoli and extra-thoracic airways. Air “trapped” in hyperinflated segments of lung may be liberated by the panting maneuver and flow to large airways; this airflow decreases measured pressure changes (as the pressure generated by panting leads to flow rather than compressed volume). Underestimating pressure changes leads to the volume of air in the lungs being overestimated.
Helium dilution is an alternative method of measuring lung volumes. The helium dilution method may be used when an individual is unable to perform plethysmography (due to dyspnea, claustrophobia, inability to perform the maneuvers, etc.) or when obstructive airways disease is present and there is concern for overestimating lung volumes. Regardless of the method used to measure lung volumes, the values directly measured include FRC, ERV, and IVC. TLC and RV may be calculated in the following fashion:
RV = FRC – ERV
TLC = RV + IVC
As with spirometry (see above), lung volumes are reported as both measured values and as a percent predicted value. Predicted values are generated from large cross-sectional studies. Determinants of predicted lung volumes include height, gender, race/ethnicity, and age. Due to continued lung growth and changes in body habitus and height, age is particularly important in determining predicted values in children and adolescents, and separate prediction equations are used for these populations. For individuals aged ≥18 years, a set of reference equations has been endorsed by the European Respiratory Society and appears to perform well in Caucasian, African-American, and Mexican-American populations. The American Thoracic Society has adopted predictive equations for subjects 8–80 years old.
At least two measurements of lung volumes should be performed regardless of the method used, and, as noted above, performing three separate measures of ERV and IVC is appropriate to account for effort-dependent variation. Lung volume measurements are considered to be reproducible if the TLC values are within 0.2 L of each other, and in that case the mean of the measured values is reported.
As with spirometry, 95% confidence intervals may be used to grade the severity of abnormalities in measured compared to predicted lung volumes (see table 36.2). Deviations from the predicted values for lung volumes can be quantified as mild, moderate, or severe in nature.
Table 36.2 USING CONFIDENCE INTERVALS TO GRADE SEVERITY OF DEFICITS IN TOTAL LUNG CAPACITY: DIFFERENCE OF PREDICTED MINUS MEASURED VALUE FOR MEN AND WOMEN
MEN | WOMEN | |
Normal: <1 confidence interval from predicted value | <1.61 L | <1.08 L |
Mild deficit: ≥1.0 to 1.5 CI from predicted value | 1.61–2.41 L | 1.08–1.61 L |
Moderate deficit: ≥1.5 to 2.0 CI from predicted value | 2.41–3.21 L | 1.62–2.15 L |
Severe deficit: ≥2.0 CI from predicted value | ≥3.22 L | ≥2.16 L |
DLCO: MEASUREMENT AND CORRECTION FACTORS
The diffusing capacity for carbon monoxide (DLCO) is a test that quantifies the diffusing properties of the alveolar-capillary membrane. The DLCO provides information about the efficiency of gas exchange at the level of the alveolus and capillary.
The DLCO is typically measured by a single-breath method. While breathing through a mouthpiece, the subject takes a breath to RV from TLC, inhaling a mixture of air that typically contains a known quantity of an insoluble gas (typically 10% of a gas such as helium, methane, argon, or neon) and 0.3% carbon monoxide (CO). The breath is then held for 10 seconds before exhaling. The first 0.75–1.0 L of the exhaled breath is discarded, as it represents dead space of the tubing and upper airways (if the FVC is <2.0 L, then the first 0.5 L is discarded.) The next 0.5 to 1 L of air is analyzed, and the quantity of the insoluble gas present in the exhaled air indicates the degree of dilution of the inhaled gas with the air already in the alveoli.
Exhaled CO is measured and corrected for dilution by gas already in the alveoli (using the known dilution of the insoluble gas). The difference between the amount of CO inhaled and the amount present in the exhaled gas (corrected for dilution) represents the uptake of CO across the alveolar-capillary membrane. By convention, DLCO is reported in units of mL/[(min) (mm Hg)], where mL indicates the volume of CO taken up across the alveolar-capillary membrane and mm Hg indicates the partial pressure of CO in the alveolus. Because the partial pressure of CO in the pulmonary capillaries is normally zero, the partial pressure of CO in the alveolus is essentially the driving pressure of CO across the alveolar-capillary membrane. To account for the length of time of the breath-hold maneuver to allow for transfer of CO across the alveolar-capillary membrane, units of time are present in the denominator. DLCO may be considered via the following equation:
DLCO = total CO uptake/(time × Paco)
The European Respiratory Society prefers to report the amount of CO taken up across the alveolar membrane in millimoles and the partial pressure of CO in kilopascals (i.e., DLCO is reported as mmol/[(min) (kPa)]).
Correcting for a subject’s hemoglobin is a more clinically relevant adjustment of DLCO, as CO binding to hemoglobin is a requisite for CO uptake across the alveolar membrane. The calculation adjusting DLCO for hemoglobin (Hb) is expressed as follows:
For men: DLCO (Hb) = DLCO × (1.7 Hb/(10.22 + Hb))
For women: DLCO (Hb) = DLCO × (1.7 Hb/(9.38 + Hb))
The units of hemoglobin in this equation are g/dL. These equations are relevant for Hb concentrations of ≥7 g/dL.
OBSTRUCTIVE VENTILATORY DEFICITS
DEFINITION AND CHARACTERISTICS
Obstructive ventilatory deficits are defined by a reduction of expiratory flow as measured by FEV1 or PEFR. Pathophysiologically, obstructive ventilatory deficits are due to increased airways resistance. Increased airways resistance results in impaired expiratory air flow. In the later stages of obstructive ventilatory diseases, incomplete exhalation due to increased airways resistance and impaired flow may result in hyperinflation and intrinsic positive end-expiratory pressure (PEEP).
There are a variety of pathologic processes that can cause obstructive ventilatory deficits, ranging from reversible air flow obstruction (i.e., asthma) to permanent changes in the lung parenchyma and airways (i.e., emphysema). Regardless of the underlying process, the primary manifestation of an obstructive ventilatory deficit is a decreased FEV1 as measured by spirometry. Typically, FVC is relatively preserved in obstructive ventilatory diseases compared to the decrease in FEV1. The relative preservation of FVC occurs as the volume of air in the lungs is not decreased, and given adequate time a patient can exhale a relatively larger quantity of air during a compete exhalatory effort than is possible in 1 second of exhalation.
In the setting of a decreased FEV1 and a relatively preserved FVC, the FEV1/FVC ratio is decreased. This constellation of findings (decreased FEV1 as compared to FVC and decreased FEV1/FVC) is the hallmark of obstructive ventilatory deficits.
As described above (see Spirometry above and Figure 36.2), the classic coved appearance of a flow-volume loop in a patient with an obstructive ventilatory deficit is due to decreased expiratory flow rates. As depicted on a flow-volume loop, the overall volume of air exhaled may not be significantly decreased compared to predicted values. As the underlying disease process progresses, FVC may decrease (e.g.,, due to progressive air trapping in emphysema), and reduced FEV1 and reduced FVC with a normal FEV1/FVC has been reported to occur in up to 10% of patients with a clinical diagnosis of COPD and normal TLC.
Lung volumes may be abnormal in certain obstructive ventilatory deficits. Particularly in emphysema, hyperinflation or air trapping may be present. Air trapping is defined by an increase in RV of >120% of predicted. Hyperinflation is defined as a TLC >120% of predicted and an RV >140% of predicted.
Lung volumes can occasionally be elevated without spirometric evidence of an obstructive deficit in normal subjects.
DIFFERENTIAL DIAGNOSIS
Any process that increases airways resistance and reduces airflow velocity in a clinically significant manner can result in an obstructive ventilatory deficit. Differentiating between reversible and irreversible processes may be a clinically useful way to approach obstructive ventilatory deficits. Bronchodilator responsiveness (discussed below) is helpful in determining the presence (or absence) of reversibility in most cases. Some pathologic processes may be “partially” reversible, thereby complicating determining the cause of obstruction.
An alternative approach to obstructive ventilatory deficits that may aid in understanding the causative pathophysiological process is to consider the structural deficit leading to airways obstruction. Specifically, abnormalities in the airway lumen, in the wall of the airway, or in the peribronchial structures may each result in an obstructive deficit.
Possible causes of an obstructive ventilatory deficit include chronic obstructive pulmonary disease (COPD) including emphysema and chronic bronchitis, as well as asthma, congestive heart failure, bronchiectasis, pneumonia, foreign body aspiration, or bronchiolitis.
GOLD CRITERIA AND CONFIDENCE INTERVALS
COPD is one of the most important and common causes of an obstructive ventilatory deficit. There are various methods for classifying severity of COPD. One of the most disseminated and widely used classification schemes is the GOLD criteria (Global Initiative for Chronic Obstructive Lung Disease).
The GOLD criteria are widely accessible and easy to use for diagnosing and grading the severity of COPD and have raised awareness about COPD (www.goldcopd.com). Although not a perfect tool for classification of severity, the GOLD criteria are useful in considering treatment and predicting prognosis for a symptomatic patient with COPD (see table 33.1 in chapter 33).
BRONCHODILATOR RESPONSIVENESS
Assessing for bronchodilator responsiveness is commonly done in the evaluation of obstructive ventilatory deficits. Bronchodilator response can be assessed in one testing session by administering a short-acting medicine or over the course of two (or more) testing sessions with interval longitudinal treatment with a bronchodilator or bronchodilators. Bronchodilator responsiveness is defined as an increase in the percentage predicted FEV1 and/or FVC of at least 12% and 0.2 L above baseline. Changes of <8% or <0.15 L are thought to be due to test-to-test variability and not due to a medication effect. Although not commonly measured as a marker of bronchodilator responsiveness, improvement in distance walked in the 6-minute walk test is another very reproducible marker of the effectiveness of bronchodilator responsiveness.
The drug, dose, and method of delivery of the bronchodilator for responsiveness assessment are not standardized. Typically when testing bronchodilator responsiveness in one testing session, a short-acting inhaled medicine is appropriate. Inhaled albuterol (four puffs of a 90-µg metered dose inhaler) through a spacer is a typical regimen. An interval of 15–20 minutes between measuring baseline spirometry and postbronchodilator spirometry values allows time for any effect of the medicine to occur. If it is of particular clinical interest, a different short-acting medication (such as ipratropium) can be used at the request of the ordering physician. Of note, one large study reported >50% bronchodilator responsiveness in patients with moderate to severe COPD when a combination of an inhaled anticholinergic plus an inhaled beta agonist was administered in one testing session.
The clinical relevance of bronchodilator responsiveness (or the absence thereof) is unknown. Although some individuals may have a correlation between bronchodilator responsiveness and symptom improvement, others may experience significant subjective improvement after treatment with an inhaled bronchodilator despite the lack of improvement in FEV1 or FVC. A possible explanation for this apparent discordance is that bronchodilators affect airway resistance and airway flow most at tidal volumes. FRC has been shown to decrease after bronchodilator therapy, which may partially explain an improved sense of dyspnea. Therefore, assessing for changes in FEV1 and FVC by forcibly exhaling from TLC (i.e., performing a FVC breath) may not adequately represent these changes at tidal volume breathing.
HOW PFTs GUIDE TREATMENT
The degree of decrease of the FEV1 can guide treatment in patients with COPD. Treatment for other obstructive ventilatory deficits may also be driven by the measured FEV1; however, clinical management based on FEV1 is most standardized for COPD.
For all patients with COPD, risk reduction is appropriate. Specifically, when applicable, encouragement of and assistance with smoking cessation is of the utmost importance. Appropriate vaccinations (influenza and pneumococcal vaccines) are appropriate for all patients with COPD. For patients with mild disease (GOLD stage 1), short-acting bronchodilators on an as-needed basis are usually considered to be sufficient. Patients with moderate (GOLD stage 2) disease should receive long-acting bronchodilators (typically long-acting beta agonists) in addition to short-acting as-needed inhalers. Inhaled corticosteroids are added to the regimen of patients with severe disease (GOLD stage 3). Finally, very severe disease (GOLD stage 4) may necessitate oxygen treatment depending on the patient’s oxygenation. Lung volume reduction surgery may be offered to a very select group of patients with primarily apical bullous disease.
The value of continued patient education is important in improving patients’ understanding of their disease. Pulmonary rehabilitation has been demonstrated to improve patients’ quality of life, although there has not been a demonstrable effect on mortality. When to refer to pulmonary rehabilitation is unclear, but the GOLD criteria advocate early (i.e., GOLD stage 1) referral.
The BODE score is a multifactorial index used to grade the severity of disease in patients with COPD. BODE is an acronym for body mass index, airflow obstruction (as measured by FEV1), symptoms of dyspnea, and exercise capacity (as measured by 6-minute walk). The BODE score has been shown to be better than FEV1 alone for predicting mortality in patients with COPD and can guide treatment.
RESTRICTIVE VENTILATORY DEFICITS
DEFINITION AND CHARACTERISTICS
Restrictive ventilatory deficits are defined by abnormally reduced lung volumes. Typically, confidence intervals around the percent predicted values of lung volumes are used to determine the degree of restriction. In restrictive diseases, lung volumes are decreased below their expected values.
Spirometry has been shown to be sensitive in ruling out restrictive ventilatory deficits; in one large retrospective study, a normal FVC was associated with restrictive disease in <3% of cases. An abnormal FVC with a normal FEV1/FVC ratio, however, was present in subjects with normal lung volumes in >40% of cases. Therefore, spirometric measurements of FVC can be considered relatively sensitive but not specific. Measuring lung volumes is necessary to confirm a restrictive ventilatory deficit when the FVC is abnormally low with a normal FEV1/FVC.
It is important to emphasize that airways resistance is not increased in restrictive ventilatory deficits caused by parenchymal diseases (such as fibrosis). In fact, PEFR may be increased compared to predicted values. This may be due to the airways being in effect “tethered” open by fibrotic changes of the lung parenchyma. Not all restrictive disease is due to pulmonary fibrotic changes, however, so an increase in PEFR, although suggestive, is not diagnostic of a restrictive ventilatory deficit.
DIFFERENTIAL DIAGNOSIS
Restrictive ventilatory deficits can be due to any process that results in decreased lung volumes. Fibrotic changes in the lung parenchyma can manifest as a restrictive deficit as the lung tissue becomes less compliant. The loss of compliance leads to lower lung volumes as a given inspiratory effort results in less inhaled volume.
Restrictive deficits can also occur from extrapulmonary processes. Pleural diseases, from pleural effusions to pleural fibrosis, can limit lung expansion and manifest as low lung volumes and a restrictive ventilatory pattern. The lungs themselves may have normal elastic properties, but the extrinsic compression from diseased pleura may limit the volume that can be inhaled.
Chest wall pathology can similarly result in a restrictive deficit. Kyphosis and/or scoliosis may result in restrictive physiology because the muscles of respiration are placed at a mechanical disadvantage. With severe curvature of the vertebral column, the diaphragm and accessory muscles may not be able to generate maximal inspiratory forces leading to decreased lung volumes. Chest wall trauma, including broken ribs or scarring (i.e., secondary to burns), may result in decreased mobility of the chest wall, extrinsic compression of the lungs, and a restrictive ventilatory deficit. Similarly, extreme obesity can cause a restrictive ventilatory deficit by a similar mechanism: extrinsic compression by excess adipose tissue can limit maximal expansion of the respiratory system.
Finally, neuromuscular diseases may cause a restrictive ventilatory deficit. Weakened or poorly functioning respiratory muscles (whether due to a myopathy or neuropathy) can limit a patient’s ability to ventilate, resulting in low lung volumes. One way of distinguishing neuromuscular disorders from other types of restrictive disease is through the use of respiratory muscle forces (often reduced) as described below. Additionally, because the ability to exhale completely to RV is an effort-dependent process, RV may actually be elevated in neuromuscular weakness.
From the above considerations, specific pathologic processes that may cause a restrictive ventilatory deficit include pulmonary fibrosis, sarcoidosis, hypersensitivity pneumonitis, medication/toxic pneumonitis, collagen-vascular diseases (i.e., scleroderma, systemic lupus erythematosus, rheumatoid arthritis), pneumothorax, pleural effusion, pleural fibrosis, severe morbid obesity, scoliosis, kyphosis, chest wall trauma or scarring, and ankylosing spondylitis. Possible neuromuscular causes of restrictive ventilatory deficits include Guillain-Barré, amyotrophic lateral sclerosis, myasthenia gravis, and muscular dystrophies.
GRADING SEVERITY
As mentioned above, confidence intervals below the predicted values of lung volumes can serve to grade the severity of a restrictive deficit. As detailed in table 36.2, the quantitative decrease of the measured TLC below the predicted value is used to grade the severity of the restrictive deficit.
Unlike obstructive deficits (COPD in particular), the severity of restrictive ventilatory deficits does not necessarily guide treatment. Treating the underlying pathologic process causing the restrictive ventilatory deficit is appropriate.
REDUCED DLCO
DEFINITION, CHARACTERISTICS, AND PHYSIOLOGY OF DECREASED DLCO
Decreased diffusing capacity for carbon monoxide (DLCO) indicates impaired diffusion of carbon monoxide (CO) across the alveolar-capillary membrane into the bloodstream and is thought to correlate with impaired diffusion of oxygen across the alveolar-capillary membrane. However, CO uptake is dependent not only on diffusion across the alveolar-capillary membrane but also on binding to hemoglobin. Therefore, the term “diffusing capacity” is inaccurate in that it implies that the transfer of CO from the alveolus to the bloodstream is dependent on diffusion alone. As such, the term transfer factor (Tlco), rather than DLCO, is used outside of the United States.
The DLCO may be conceptualized through the following equations:
DLCO = total CO uptake/(time × PACO)
or
DLCO = Vco/(PACO – Pcco)
where Vco is the uptake of CO (mL CO/min), Paco is the alveolar pressure of CO, and Pcco is the average pulmonary capillary partial pressure of CO. The Pcco is usually zero in nonsmokers.
As with measurements of spirometry and lung volume, confidence intervals are used to grade the severity of reductions in DLCO. Reductions in percentage predicted of DLCO can also be used to grade severity. Table 36.3 delineates a schema for grading the severity of reductions in DLCO.
As described above, the DLCO may be “corrected” for hemoglobin level. This is a physiologically meaningful correction, as CO uptake is directly dependent on blood hemoglobin concentration. Decreases in DLCO corrected for hemoglobin concentration [DLCO (Hb)] may be graded with confidence intervals.
CLINICAL UTILITY AND MEANING OF DLCO
The DLCO can provide useful information about the functional relationship of the alveoli and the pulmonary capillaries. By extension, the DLCO may provide some information about gas exchange in general. Given similarities of the mechanism of CO uptake (diffusing across the alveolar membrane and being taken up by hemoglobin) to oxygen uptake, clinicians extrapolate the results of DLCO measurements to oxygen uptake. When DLCO is interpreted in this fashion, it at best provides a rough guide of actual oxygen diffusion and uptake.
DIFFERENTIAL DIAGNOSIS
DLCO may be decreased or increased as compared to its predicted value. As with spirometry and lung volumes, the predicted value of DLCO is determined by equations that take into account age, height, gender, and ethnicity/race. The grade of severity of the difference in the measured DLCO from the predicted DLCO is determined by confidence intervals (see table 36.3).
Table 36.3 USING CONFIDENCE INTERVALS TO GRADE SEVERITY OF DECREASES IN DLCO: DIFFERENCE OF PREDICTED MINUS MEASURED VALUE FOR MEN AND WOMEN
MEN | WOMEN | |
Normal: <1 confidence interval from predicted value | <7.99 | <6.50 |
Mild deficit: ≥1.0–1.75 CI from predicted value | ≥7.99–13.98 | ≥6.5–11.37 |
Moderate deficit: ≥1.75–2.5 CI from predicted value | 13.99–19.97 | 11.38–16.24 |
Severe deficit: ≥2.5 CI from predicted value | ≥19.98 | ≥16.25 |
NOTE: Units are in mL/[(mm Hg)(min)].
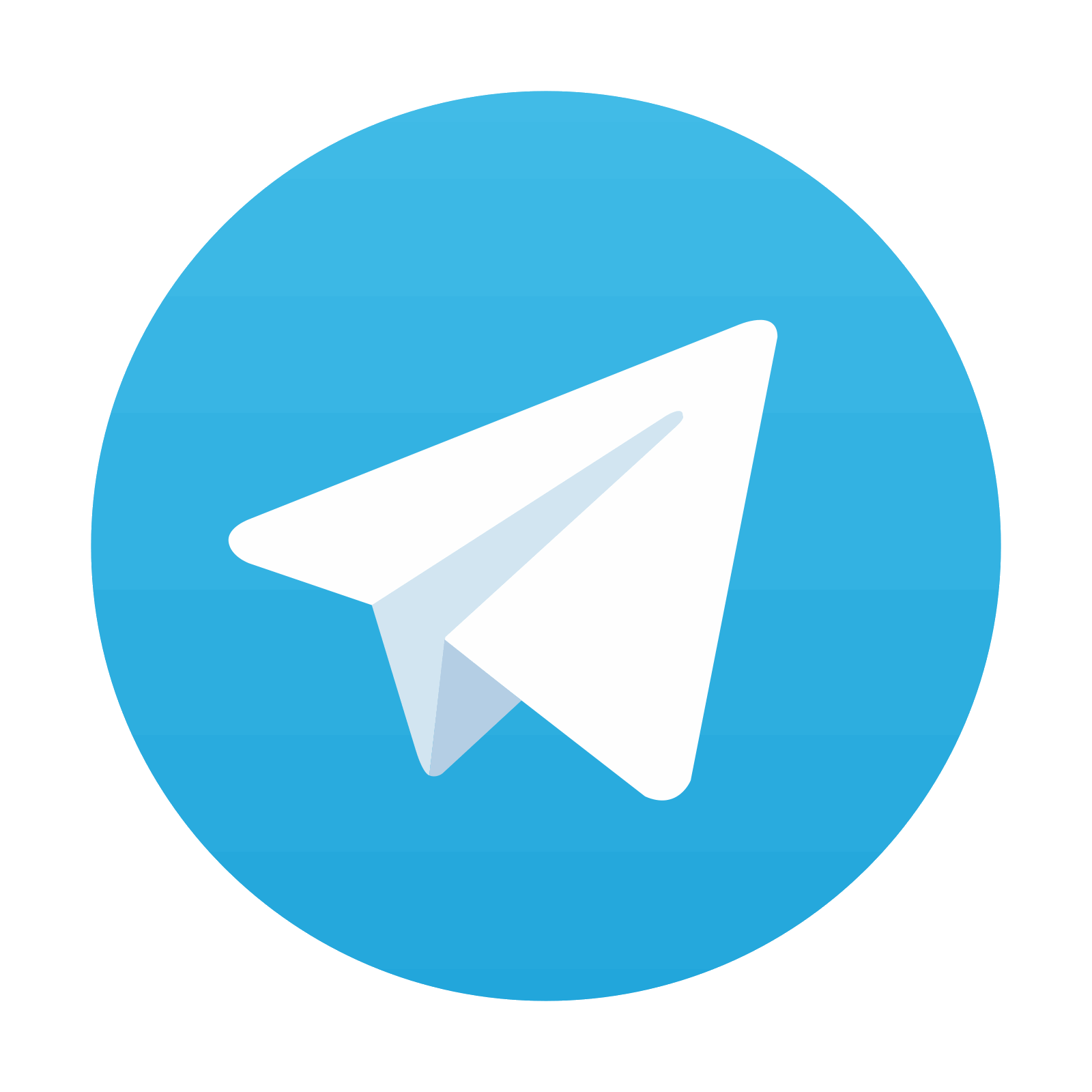
Stay updated, free articles. Join our Telegram channel
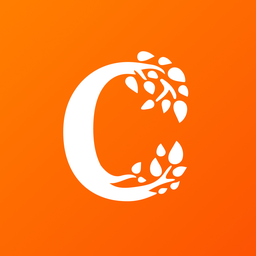
Full access? Get Clinical Tree
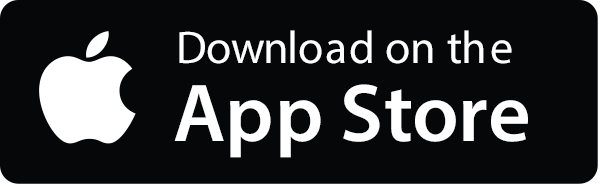
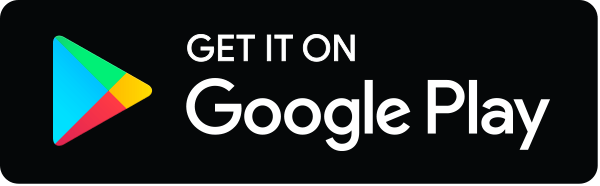