CONTENTS
11.2 Anatomy and Physiology of the Respiratory Tract: Implications for Pulmonary Drug Delivery
11.2.2 Mucus and the Mucociliary Escalator
11.3 Overview of Pulmonary Drug Delivery
11.3.1 Limiting Factors for Pulmonary Drug Delivery
11.3.1.2 Mucus and Mucociliary Clearance
11.3.1.7 Patient Acceptability and Compliance
11.4 Particle Deposition in the Airways
11.4.1 Mechanisms of Inhaled Particle Deposition in the Airways
11.4.2 Pharmaceutical Factors Affecting Particle Deposition
11.4.3 Drug Factors Affecting Particle Deposition
11.4.4 Physiological Factors Affecting Particle Deposition
11.4.5 Pathological Factors Affecting Particle Deposition
11.5 Endotracheal Instillation
11.7.1 CFC to HFA Propellant Transition
11.7.4 Future Directions in pMDIs
11.8.1 DPIs for Local Lung Action
11.8.2 DPIs for Systemic Delivery
11.12 Conclusions and Future Directions
Historically, inhalation aerosol therapy dates back to ancient times when the ancient Greeks (including Hippocrates) and Egyptians used “vapors” to treat asthma and tuberculosis (TB) (Murphy 2007; O’Callaghan et al. 2002; Patton and Byron 2007). In 1778, an English physician by the name of John Mudge invented his own pewter tank inhaler for the treatment of a cough (Sanders 2007). Starting in the early twentieth century, bronchodilators and biologics (antibiotics and insulin) were commonly being delivered via inhalation devices (Newman et al. 2009). Vast advancements have recently been made in inhalation therapy. In 2013, the global pulmonary drug delivery market reached $32.4 billion and is expected to reach $43.9 billion in 2018 (Marketwatch 2014).
Pulmonary drug delivery is primarily used to treat local conditions of the airways, thereby delivering drugs directly to their site of action, for the treatment of respiratory disorders such as asthma and chronic obstructive pulmonary disease (COPD). Such drugs include, for example, short-acting β-agonists (e.g., albuterol), short-acting corticosteroids (e.g., beclomethasone), short-acting anticholinergics (e.g., ipratropium), mast cell inhibitors (e.g., cromolyn sodium), long-acting β-agonists (e.g., salmeterol and formoterol), long-acting corticosteroids (e.g., fluticasone propionate), and long-acting anticholinergics (e.g., tiotropium). Pulmonary drug delivery is also used for the local delivery of destructive mucolytics (e.g., dornase alpha) and nondestructive mucolytics (e.g., hypertonic saline solution and mannitol) in the treatment of cystic fibrosis (CF), as well as antibiotics (e.g., tobramycin and aztreonam) and antivirals (e.g., ribavirin and zanamivir) for respiratory infections.
An emerging application of pulmonary drug delivery is for systemic delivery, providing a painless, needle-free, noninvasive route of entry for the drug to the blood; very rapid absorption is possible. The pulmonary route is showing considerable promise for the systemic delivery of small molecules but also macromolecules like proteins, peptides, and vaccines, as well as gene-based therapies. Both local and systemic drug delivery applications are discussed in this chapter. The invasive method of endotracheal instillation, for localized lung delivery, is also described.
Given the multifactorial nature of pulmonary diseases, combination drug aerosol products (containing drugs from different therapeutic classes in the same aerosol) continue to grow and are the top-selling aerosol products worldwide. For superior disease state management and prevention, codeposition of inhaled pulmonary drugs in the same aerosol to the lung has been shown clinically to be superior than inhaling two separate single-drug aerosol products where codeposition of both drugs does not occur. In addition, the aerosol treatment burden on the diseased lungs is less with a combination drug aerosol product. Currently, several dual-drug combination aerosol products containing two drugs from two different therapeutic classes are commercially available as liquid aerosols and inhalable dry powders for the treatment and management of airway inflammatory disorders, including asthma, CF, and COPD. These top-selling dual-drug combination inhalation products are DuoNeb®, Advair®, Spiriva®, Dulera®, and Symbicort®. The upcoming sections will present these aspects in detail.
This chapter will describe pulmonary drug delivery in the context of treatment of human lung diseases and approved respiratory products used by patients. In addition, the different inhalation aerosol devices approved for human use and respiratory pharmaceutical products will be described. Finally, future trends and outlook will be presented.
11.2 ANATOMY AND PHYSIOLOGY OF THE RESPIRATORY TRACT: IMPLICATIONS FOR PULMONARY DRUG DELIVERY
The anatomy of the respiratory system is shown in Figure 11.1. The respiratory tract begins at the nose (drug delivery to the nasal cavity is the focus of Chapter 10). The nasal passageways open into the pharynx (throat), which serves as a common passageway between the respiratory and digestive systems. The larynx (voice box, which houses the vocal cords) is located at the entrance of the trachea. Beyond the larynx, the trachea (windpipe) divides into the left and right primary bronchi, which enter the left and right lungs, respectively. Upon entering the lungs, the primary bronchi divide to form the secondary (lobar) bronchi, which in turn branch into the tertiary (segmental) bronchi and then the bronchioles. These further branch repeatedly, forming a fine network of bronchioles, which end in the terminal bronchioles. Each successive branching results in progressively narrower, shorter, and more numerous airways; the overall effect resembles an inverted tree, described as the bronchial tree. Clustered at the ends of the terminal bronchioles are the alveoli, tiny air sacs where gas exchange between air and blood takes place (see Chapter 4, Figure 4.10).
FIGURE 11.1 Anatomy of the lungs. (Detail of the alveoli, Alex Mit/Shutterstock.com.)
Anatomically, the respiratory tract can be divided into two sections (Tortora and Derrickson 2013), as shown in Figure 11.1: (1) the upper respiratory system, which includes the nose, pharynx, and associated structures; and (2) the lower respiratory system, which includes the larynx, trachea, bronchi, and lungs. Functionally, the respiratory tract can also be divided into two parts: (1) The conducting zone is a series of interconnected cavities and passageways, which includes the nose, pharynx (throat), larynx, trachea, bronchi, bronchioles, and terminal bronchioles. These serve to prepare ambient air for respiration by warming and humidifying the incoming air, as well as filtering the air of foreign particles and pathogens. (2) The respiratory zone is the tissues within the lungs where gas exchange between air and blood occurs. These tissues include the respiratory bronchioles, alveolar ducts, alveolar sacs, and alveoli.
Specific anatomical and physiological features of the respiratory system have important implications for pulmonary drug delivery and are discussed in more detail here.
The basic structure of the respiratory tract can be considered as a series of dichotomous branches (Figure 11.1). Each time the bronchial tree divides, it is referred to as a new generation; thus, the trachea (generation 0) divides into the primary bronchi (generation 1), then the secondary bronchi (generation 2), etc. There are 23 generations of airways; gas exchange occurs in the last 8.
This branching of the bronchial tree is associated with a number of key features. First, airway branching causes a reduction in the airflow velocity. Within the bronchial tree, airflow is highest in the bronchi and rapidly decreases as it descends into the lower airways. Airflow velocities in the main bronchi are estimated to be 100-fold higher than in the terminal bronchioles and 1000-fold higher than in the alveoli. In the alveoli, the air velocity is near zero, to facilitate respiration by passive diffusion of dissolved gases. Second, with the increasing number of bifurcations, the total cross-sectional area is increased significantly, so that the overall surface area of the alveolar region is very large. Third, the branching of the bronchial and bronchiolar tree is associated with significant changes in the epithelial lining, as described in Section 11.2.3.
11.2.2 MUCUS AND THE MUCOCILIARY ESCALATOR
With the exception of the alveolar region, mucus is secreted in the respiratory tract by goblet cells in the mucosa, as well as by submucosal glands. The mucus of the respiratory tract has already been described for nasal drug delivery (Chapter 10, Section 10.2.2). A ciliated epithelium lines most of the airways and is described in detail in Chapter 4 (Section 4.5.4). Each ciliated cell has about 200 cilia, as well as many interspersed microvilli. The cilia are surrounded by a low-viscosity fluid, known as the periciliary fluid layer; they project into the more viscous, overlying, mucus layer. As described in Chapter 10 (Section 10.2.2), mucus functions as a protective barrier against the entry of pathogens and also helps to filter the incoming air via the “mucociliary escalator.” Dust particles, microbes, allergens, and other pollutants become trapped in the viscoelastic mucus layer. Rhythmic beating of the cilia propels the mucus, with any entrapped pollutants, against gravity toward the throat. The ciliary beat frequency is in the range of 1000–1200 beats/min. At the throat, entrapped particles are then either swallowed or expectorated, thereby removing them from the air-ways. Coughing and sneezing speed up the movement of cilia and mucus, accelerating the removal process. Soluble particles can also dissolve in mucus and may then be removed via absorption from the airways. A further lung clearance mechanism involves phagocytosis by alveolar macrophages (see Section 11.3.1.4).
As the branching becomes more extensive in the bronchial tree, the respiratory epithelium undergoes considerable structural changes, as outlined in Table 11.1 (see also Chapter 4, Figures 4.9 and 4.10). In summary, the thick, pseudostratified, ciliated epithelium (with overlying mucus) found in the upper airways gradually gives way to a progressively thinner epithelium with less mucus and cilia in the bronchioles and eventually becomes an extremely thin, nonciliated, single layer of squamous cells, with no overlying mucus layer, at the level of the alveoli.
Respiratory Region |
Epithelium |
Primary, secondary, and tertiary bronchi |
Pseudostratified ciliated columnar epithelium, with many goblet cells (see Chapter 4, Figure 4.9) |
Larger bronchioles |
Ciliated simple columnar epithelium with some goblet cells |
Smaller bronchioles |
Ciliated simple cuboidal epithelium with no goblet cells |
Terminal bronchioles |
Nonciliated cuboidal epithelium |
Alveoli |
Simple squamous epithelium (see Chapter 4, Figure 4.10) |
The structure of the alveoli has been described in detail in Chapter 4 (Section 4.5.4.3 and Figure 4.10). The walls of the alveoli consist of two types of epithelial cells: (1) type 1 alveolar cells, comprising simple squamous epithelial cells, are the main sites for gas exchange. Type 1 cells cover 90% of the entire alveolar surface. (2) Type 2 alveolar (also called septal) cells secrete alveolar fluid, which comprises a lung surfactant monolayer on a thin aqueous layer. Pulmonary surfactant lowers the surface tension within the alveoli, thereby maintaining alveolar structural integrity. Also associated with the alveolar wall are the alveolar macrophages, which account for about 3% of the cells in this region.
11.3 OVERVIEW OF PULMONARY DRUG DELIVERY
As outlined in Section 11.1, the respiratory system can be used both for localized drug delivery to the lungs and as a noninvasive route for systemic delivery. Drugs used to exert a local effect, such as anti-asthmatics, anti-inflammatories, and mucolytics, are delivered directly to their site of action in the airways. Thus, therapeutic agents are effectively targeted to their site of action, maximizing therapeutic effect. Lower doses are therefore required for effective therapy, reducing the risk of systemic side effects and toxicity, as well as costs. By delivering the drug directly to the site of action, a more rapid onset of action is also possible, in comparison to the oral route.
For systemically acting drugs, pulmonary delivery also offers a number of potential advantages:
1. An expanded surface area: Physiologically, the lungs are designed for the efficient exchange of respiratory gases. The extensive branching results in a large total cross-sectional area. The surface area of the adult lung is in the range of 80–150 m2, equivalent to the surface area of a tennis court. In addition, it is estimated that the lungs contain 300 million alveoli for gas exchange. These features also offer great potential for the delivery of systemically acting drugs.
2. Thin and highly permeable epithelium: The alveolar epithelial membrane is very thin and permeable, to facilitate its physiological role of rapid passive gas exchange (see also Chapter 4, Figure 4.10). A network of capillaries spreads over the outer surface of the alveoli (Figure 11.1). The exchange of oxygen and carbon dioxide takes place by gas diffusion across the alveolar and capillary walls, which together form the respiratory membrane, which comprises merely: a single epithelial cell (i.e., a type 1 pneumocyte), a single endothelial cell (which lines the blood capillary), and a common basement membrane between the two (see also Chapter 4, Figure 4.10). The respiratory membrane is at least an order of magnitude thinner than other epithelial membranes, such as in the intestines, and several orders of magnitude thinner than, for example, the skin epidermis. Thus, the alveolar epithelium has a higher (in many cases, much higher) permeability than alternative mucosal routes, which facilitates the absorption of large molecular weight APIs (including insulin), which are usually too large to permeate other epithelial interfaces. Drug absorption from this region is also very rapid, so that rapid onset of therapeutic activity is possible. The epithelium of the higher airways, such as the trachea and bronchi, is thicker than the alveolar region, but is still very thin in comparison to other epithelial interfaces and these regions also demonstrate enhanced permeability.
3. Rich blood supply: The lungs receive blood via the pulmonary arteries (deoxygenated blood) and the bronchial arteries (oxygenated blood from the aorta). The network of capillaries that covers the alveoli is so dense that each alveolus is effectively encircled by an almost continuous sheet of blood. This highly vascular surface further promotes the rapid absorption of drug molecules and ensures a rapid onset of action.
4. Relatively low-enzymatic activity: The enzymatic activity associated with respiratory secretions is considerably lower than that of the GI tract. Furthermore, drugs absorbed via the respiratory route avoid the intestinal and hepatic first-pass metabolism associated with oral drug delivery. Pulmonary drug delivery is therefore an attractive option for enzymatically labile drugs, such as therapeutic peptides, proteins, and DNA-based medicines.
5. Accessibility and compliance: As described later, a large number of different types of inhalable devices allow for the relatively easy self-administration of drugs, for either local or systemic effects. The pulmonary route offers a painless, needle-free, noninvasive alternative to injections for systemic drug delivery.
11.3.1 LIMITING FACTORS FOR PULMONARY DRUG DELIVERY
The anatomy and physiology of the lungs also afford a number of obstacles to successful drug delivery. As described in Section 11.6 et seq, pulmonary drug delivery is typically achieved via the administration of an aerosol, i.e., a suspension of fine solid or liquid particles, dispersed in a gas. The respiratory system is characterized by a variety of efficient defense mechanisms to both minimize the entrance of particulates into the airways and ensure the effective clearance of any particles that have been deposited. Although these mechanisms protect the airways from the entrance of contaminants such as dust, fumes, pollutants, and pathogens, they also present significant barriers to effective drug delivery. These, and other, barriers to pulmonary drug delivery are described here.
The branching architecture of the airways is such that it is very difficult for drug molecules to access and penetrate deep into the lungs. To travel down the airways, the drug particles (which are often traveling at high speeds) must pass through a successive series of branching tubes, of progressively decreasing size. As described in Section 11.2.1, there are about 23 generations of branching, en route to the alveolar space. Once the aerosol droplet/particle has deposited in the airways, there are a number of further obstacles to be surmounted, as described next.
11.3.1.2 Mucus and Mucociliary Clearance
The mucus layer and the process of mucociliary clearance present a number of obstacles for pulmonary drug delivery. Drugs delivered as dry powders must first dissolve in the mucus secretions. Typically, the high water content and the presence of surfactant (i.e., fatty acids and phospholipids) in mucus aid the dissolution process. However, dissolution can be an issue for poorly soluble APIs such as the anti-inflammatory corticosteroids, which are often poorly soluble and administered as dry powders.
The mucus also presents a significant diffusional barrier for a drug in transit to the epithelial surface. The thickness of the mucus layer is about 5–8 μm in the larger airways (although this can increase significantly in disease states, see below), then it progressively decreases in thickness, until it disappears in the respiratory region. The rate of diffusion of an API through mucus depends on a number of factors, including the thickness and viscosity of the mucus layer, as well as the physicochemical properties of the drug. Interactions between the API and the mucus layer may also occur, leading to mucoadhesion, via hydrogen bonding; charge interactions can also occur, between a positively charged drug and negatively charged sialic acid residues of the mucus layer.
Mucociliary clearance may limit the contact time of a drug molecule with the epithelial surface. The drug, instead of settling locally, can instead be removed to the throat, to be swallowed or expectorated. Limited contact time with the absorbing surface can compromise drug absorption for a systemically active drug, or shorten drug–receptor interaction time for a locally acting drug.
As can be seen from Table 11.1, on penetrating deeper into the airways, the epithelium presents a progressively thinner diffusional barrier. Also, mucociliary clearance declines as the number of ciliated cells decreases and less mucus is produced. At the level of the alveoli, the epithelium comprises merely a single layer of highly permeable squamous cells, with no overlying mucus layer, or mucociliary clearance process. This region is also characterized by very large surface area and rich blood supply. For these reasons, delivery systems designed for systemically acting drugs should target the alveolar region. However, gaining access to this region is difficult, due to the various barriers and clearance mechanisms present.
As described in detail in Chapter 4 (Section 4.3), drug absorption across any epithelial interface can be via the paracellular route (i.e., between epithelial cells), or the transcellular route (i.e., through the cells). The presence of tight junctions between epithelial cells significantly limits paracellular transport, typically confining transit via this route to small hydrophilic molecules such as mannitol. There is evidence that the tight junctions of alveolar epithelium are leakier than at other epithelial interfaces, so that more paracellular transport is known to occur in this region (Chandra et al. 1992).
In the larger airways, the predominant pathway across the respiratory epithelium is via transcellular passive diffusion. The rate of absorption is determined by the physicochemical properties of the drug, as well as the drug concentration gradient across the cells. The various drug physicochemical factors that affect transepithelial transport have been described in Chapter 4 (Section 4.3); to summarize here, important drug properties include (1) molecular weight: large molecules (greater than 500 Da) demonstrate poor permeation, (2) lipophilicity: lipidic drugs exhibit good permeation properties, whereas polar, hydrophilic, or ionized molecules demonstrate poor permeability, and (3) drug solubility: a drug must dissolve in the respiratory fluids and be in solution for epithelial absorption to occur.
Pulmonary drug delivery may also result in absorption of an API from the GI tract, either due to direct swallowing of inhaled dose that deposits in the mouth and back of the throat, or due to secondary swallowing: drug deposited in the airways is moved, via mucociliary clearance and coughing, to the throat where it is then swallowed.
The alveolar macrophages represent a further clearance mechanism that exists in the lungs. These phagocytic cells are found in the lumen of the alveolar sacs and remove foreign particulates (e.g., fine dust, viruses, mycobacteria such as in TB, and bacteria causing pneumonia) via the process of phagocytosis (see also Chapter 4, Figures 4.4 and 4.10). Large molecular weight drugs, drug particles, and nanoparticulate drug delivery systems (DDS) can also be phagocytosed via these cells. Following phagocytosis, the drug is internalized within a phagocytic vesicle (phagosome), which subsequently fuses with a lysosome, which may lead to destruction of the API on exposure to lysosomal enzymes. An important consequence is that in order to avoid phagocytic capture, a macromolecular drug (or nanoparticulate DDS) must dissolve quickly in the alveolar fluid and be absorbed systemically.
While the lung is regarded as a low metabolic organ compared to the hepatic system and GI tract, a low number of metabolizing enzymes (e.g., phospholipases, esterases, and cytochrome p450) and transporters (e.g., p-glycoprotein) have been discovered in certain types of pulmonary cells (Bend et al. 1985; Devereux et al. 1989). This enzymatic activity could be detrimental to the absorption of enzymatically labile drugs.
Drug delivery to the lungs is a complex process and dependent on many interrelated elements, including pharmaceutical, drug, physiological, and pathological factors. This introduces a variability that may be particularly problematic for drugs with a narrow therapeutic index.
11.3.1.7 Patient Acceptability and Compliance
Aerosol devices can be difficult and unpleasant to use, which can compromise patient compliance. These difficulties are described later, with respect to each type of device, and include issues such as the difficulty in coordinating actuation with inhalation for pressurized metered-dose inhalers (pMDIs), the unpleasantness of the “cold Freon” effect (the cold blast of pMDI propellant hitting the back of the throat) for pMDIs, the long inhalation times required for nebulizer therapy, the cumbersomeness and lack of portability of nebulizer devices, the high inspiratory effort required to generate an effective aerosol for dry powder inhalers (DPIs), and the unpleasantness of dry powder hitting the back of the throat for DPIs.
11.4 PARTICLE DEPOSITION IN THE AIRWAYS
The site of particle deposition is a crucial factor affecting pulmonary drug delivery and is considered in detail here.
11.4.1 MECHANISMS OF INHALED PARTICLE DEPOSITION IN THE AIRWAYS
Particles deposit in the airways by five modes of deposition mechanisms (Altiere and Thompson 2007; Hickey and Thompson 2004; Stocks and Hislop 2002), which are comprised of three major modes and two minor modes. The three major modes are the following: (1) inertial impaction due to inertial force, (2) sedimentation due to gravitational force, and (3) diffusion due to Brownian motion. The two minor modes are: (1) electrostatic attraction due to attractive coulombic forces and (2) interception. The three major modes are described here:
Inertial impaction: Large particles, traveling at high velocity, tend to collide with the airway walls in the upper airway regions. Due to particle inertia, the particles continue on their original course and are unable to follow the sharp change in direction of the inspired air, as it weaves its way through the extensive branching of the oropharyngeal and bronchial tree. Thus, the particles impact on the airway walls, with impaction usually occurring near the sharp-angled bifurcations. For rapidly traveling particles of large size, inertial impaction is particularly relevant in the oropharyngeal region. This is as a result of the sharp change in airflow direction from the mouth to throat region, which is a ≈90° angle (Figure 11.1).
Inertial impaction is dependent on particle momentum, which in turn is dependent on particle size and velocity. Particles with larger diameters, higher densities, and higher velocities, will result in greater impaction. These properties are in turn critically influenced by the type of device used to generate the aerosol, as discussed later.
Sedimentation: Sedimentation is seen with smaller airborne particles that succumb to gravity, i.e., those particles that have successfully transited the upper airways, to reach the bronchiolar and alveolar regions. In these regions, the airstream velocity is relatively low and so deposition is primarily the result of sedimentation due to gravitational settling. The percentage of particles that deposit via this mechanism is dependent on the amount of time the particles are in these regions. If the time between the end of inspiration and the start of exhalation is extended, there is more time for sedimentation to occur. Therefore, introducing a breath-hold step after inspiration is an important mechanism to optimize the administration of many types of aerosol.
Brownian motion: Much smaller-sized particles (less than 500 nm) can deposit due to Brownian motion. The random bombardment of gas molecules can result in particle collision with the airway walls and thus particle deposition. Deposition due to Brownian motion is particularly associated with the alveolar spaces, where air velocity is near zero. It is of interest to note that inhaled cigarette smoke particles are typically in the aerodynamic size range of approximately 100–200 nm. These particles readily deposit in the alveolar region for fast systemic absorption, facilitating rapid nicotine action on the central nervous system (CNS).
11.4.2 PHARMACEUTICAL FACTORS AFFECTING PARTICLE DEPOSITION
Particle deposition is influenced by the type of delivery device used and the specifics of the drug formulation, as these factors affect aerosol velocity, as well as the aerodynamic particle size, aerodynamic particle size distribution, particle shape, particle surface charge, particle density, and hygroscopicity of the inhaled particles. Here, we will discuss two important pharmaceutical variables, namely, aerosol velocity and aerodynamic particle size. Further information is given in the discussion later with respect to each type of aerosol device.
With regard to aerosol velocity, aerosols generated by pMDIs comprise particles of large size with extremely high velocities (up to 100 km/hour), which can lead to extensive impaction in the oropharyngeal region. Estimates have suggested as much as 80% of the dose from a pMDI can be lost to oropharyngeal impaction (Dolovich et al. 1981). The aerosol particles produced by DPIs travel within the inspired air, and as such, their velocity is determined by the inspiratory flow rate (IFR) (which can vary considerably, particularly in disease states). The generation of an aerosol via a nebulizer is not dependent on the patient’s IFR, and these devices produce smaller liquid aerosol droplets with less oropharyngeal deposition. Soft mist inhalers (SMIs), by definition, produce a slow-moving aerosol mist without a propellant. The liquid aerosol is generated independently of the patient’s IFR and is associated with less oropharyngeal deposition than propellant-based liquid aerosol systems.
Aerodynamic particle size is another important determinant of particle deposition in the airways. Aerosol particle size is expressed as aerodynamic diameter (Da), defined as the equivalent diameter of a spherical particle of unit density having the same settling velocity from an air stream as the particle of interest (Suarez and Hickey 2000). It is an important measured parameter required by federal regulating agencies for the approval of any inhaled therapeutic aerosol product to be used in humans. Da has been used for several decades to quantify an aerosol particle’s inherent propensity to deposit in the lungs (Edwards et al. 1998).
Particles with Da ≥ 10 μm will not enter the lungs, but will instead exhibit extrathoracic deposition in the oropharyngeal region (Dalby et al. 2007; Murphy 2007; Suarez and Hickey 2000). Oropharyngeal impaction results in drug wastage: drug deposited here is either swallowed or expectorated and is not available to exert its therapeutic effect. It can cause local side effects, for example, the oropharyngeal candidiasis associated with inhaled corticosteroid use. Drug deposition here also feels unpleasant, can induce a cough reflex, and adversely affect patient compliance. Particles with Da < 10 μm can readily enter the lungs and exhibit thoracic deposition. Particles with Da ≤ 5 μm can readily reach the mid-lung region and smaller airways. Particles with Da ≤ 2 μm can readily reach the respiratory bronchiolar and alveolar regions.
Aerosol dispersion performance by in vitro inertial impaction testing is required for the approval of inhalation aerosol products, as described by the U.S. Pharmacopeia (USP) Chapter <601>. The aerosol dispersion performance parameters that are required are as follows (United States Pharmacopeia 2006; Xu et al. 2011):
• The emitted dose is the total mass of drug emitted from the device.
• The fine particle fraction (FPF) is the mass fraction of aerosol particles that is less than a certain aerodynamic cutoff size to the total mass of particles emitted from the device. The aerodynamic cutoff size used in calculating FPF is at the discretion of the scientist carrying out the measurements, as it depends on the type of inertial impactor used, testing conditions such as airflow, and the specific lung disease of interest.
• The mass median aerodynamic diameter (MMAD) is a calculated statistical population value based on the entire aerodynamic size distribution based on mass, below which 50% of the total aerosol mass falls under.
Taking into account the effects of aerodynamic particle size on regional lung deposition patterns, it can be seen that MMAD ≤ 5 μm of the aerosol is desirable, to facilitate predominant deposition targeted to the smaller airways. Aerosols with larger MMADs will deposit higher in the respiratory tract. It is important to note that the MMAD is a calculated statistical value based on the entire aerosol size distribution plotted on a log–probability scale. The underlying assumption is that the mass-weighted aerodynamic particle size distribution data is log-normally distributed. The population variability spread of MMAD is described by the geometric standard deviation (GSD), which is also determined from the same plot as the MMAD. An aerosol with a GSD < 1.22 is described as monodisperse, whereas a GSD 1.22 is polydisperse. A polydisperse aerosol (GSD 1.22) will also show greater deposition than a monodisperse aerosol of equivalent MMAD.
The efficacy of an aerosol device to target the airways is of course related to the aerodynamic particle size generated by the device and the formulation physicochemical properties. Different types of aerosol-generating devices generate different MMAD values, for a given formulation. Droplets leaving pMDIs can be too large with a high velocity, which tends to cause unwanted oropharyngeal deposition in the mouth and throat via inertial impaction.
11.4.3 DRUG FACTORS AFFECTING PARTICLE DEPOSITION
Particle deposition is also affected by the physicochemical properties of the drug, such as its molecular weight, solubility, lipophilicity, and charge. These factors can affect stability in vitro, for example, a hygroscopic drug, formulated as a dry powder, tends to aggregate, which can make the generation of a dry powder aerosol more difficult. Drug interactions with mucus can affect residence time and the ability of a drug to permeate through the mucus layer. Drug properties will also influence the drug’s ability to be absorbed across the respiratory epithelium, with low molecular weight, lipophilic drugs showing enhanced absorption properties (Section 11.3.1.3). For pMDIs, the importance of drug solubility in the formulation was highlighted by the difficulties encountered on switching from CFA to HFA propellants, as many drugs were insoluble in the new systems.
11.4.4 PHYSIOLOGICAL FACTORS AFFECTING PARTICLE DEPOSITION
Physiological factors affecting particle deposition include features such as the IFR, breathing patterns, thickness of the mucus blanket, mucociliary clearance, lung architecture, and patient skills. Increasing the IFR will increase airstream velocity, which will in turn increase deposition via inertial impaction in the upper airways. It will also increase airstream turbulence, which further enhances deposition, especially in the oropharyngeal region and the first few generations of the bronchial tree.
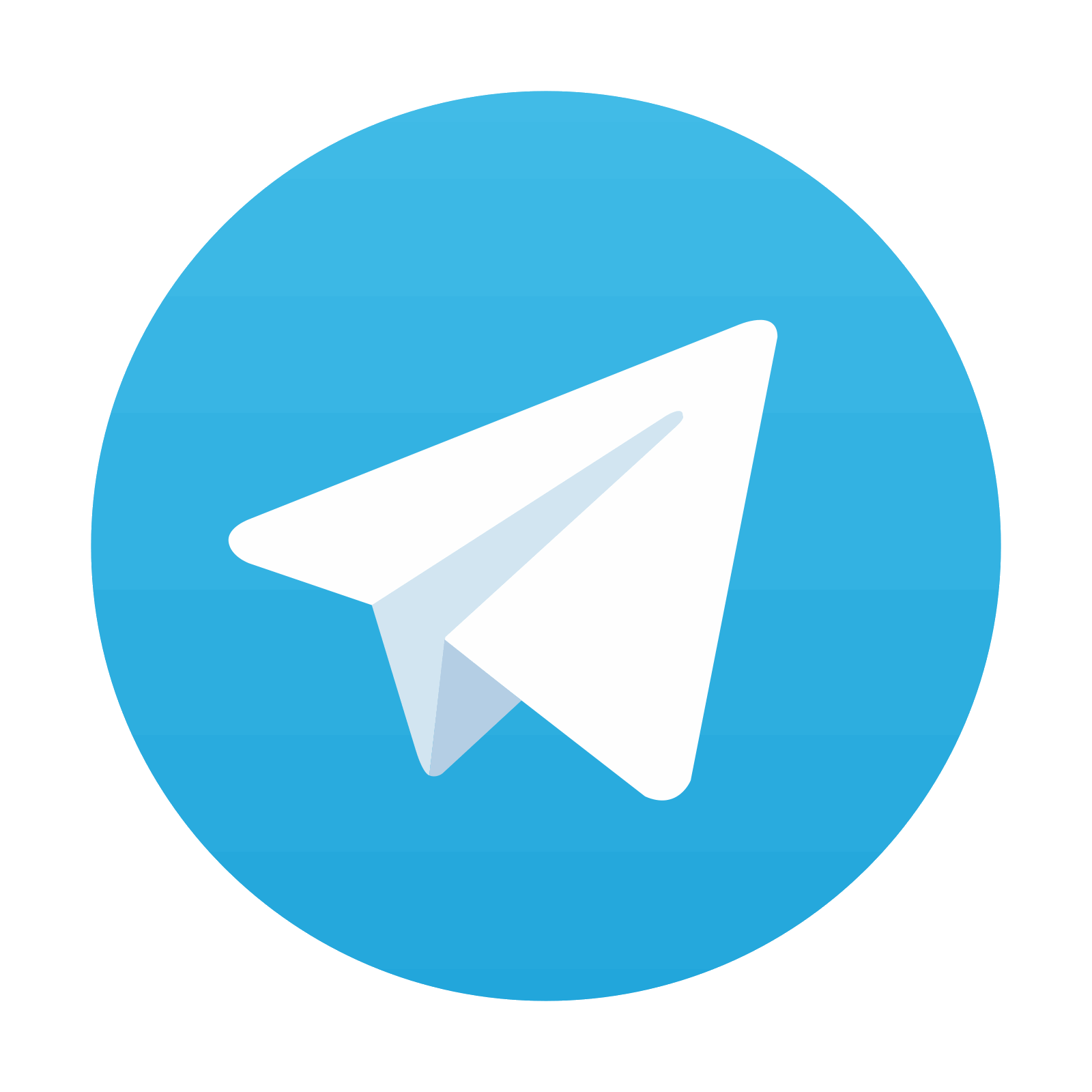
Stay updated, free articles. Join our Telegram channel
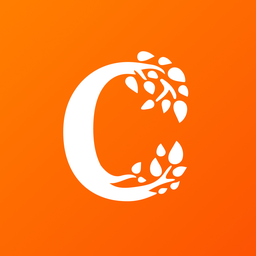
Full access? Get Clinical Tree
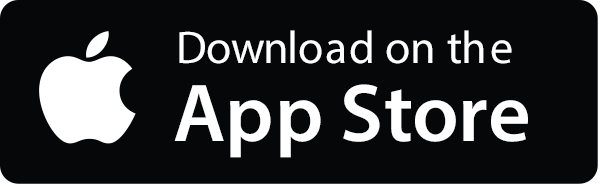
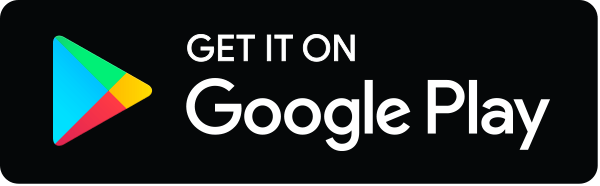