CONTENTS
18.2 Medical Imaging Modalities
18.3 Nanocarriers for Theranostics
18.4 Inorganic-Nanosized Theranostics
18.4.1 Gold Nanoparticle–Based Theranostics
18.4.2 Iron Oxide Nanoparticle–Based Theranostics
18.4.3 Quantum Dots Theranostics
18.4.4 Mesoporous Silica Nanoparticles
18.4.5 Calcium Phosphate Nanoparticles
18.5 Organic-Nanosized Theranostics
18.5.3 Polymeric Nanoparticles
18.6 Multifunctional-Nanosized Theranostics for Photodynamic Therapy
18.7 Conclusions and Future Outlook
The field of theranostics (a portmanteau of “therapeutics” and “diagnostics”) aims to integrate therapeutics with diagnosis, in order to develop more individualized therapies. Life-threatening diseases, particularly high-risk cancers, are highly heterogeneous, so that treatments are typically effective for only limited patient populations and at certain stages of disease development. Merging of the paradigms of diagnosis and therapy will provide timely assessment of therapeutic response, allowing optimization of treatments and tailoring personalized medicine based on individual needs, to improve therapeutic outcomes. A current clinical example is the use of Herceptin® (a monoclonal antibody used to treat patients with breast cancer), which is used in conjunction with the diagnostic tool, HercepTest®. Herceptin® targets the HER2 protein, which is overexpressed in approximately one-third of breast cancers. HercepTest™ specifically demonstrates overexpression of the HER2 protein in breast cancer tissues and so is used to identify those patients who are most likely to benefit from Herceptin® treatment.
Currently, research activities on theranostics are predominantly focused on cancer diagnosis and treatment. Cancer is the third most common cause of death in the world, following cardiovascular and infectious diseases. There are significant challenges in the successful delivery of cytotoxic drugs specifically to tumor cells while avoiding normal healthy tissues and minimizing side effects. In addition, it is essential to carry out diagnostic imaging to understand the cellular phenotypes, biological activity, and heterogeneity of each tumor. In response to these challenges, theranostics offers the potential to allow physicians to monitor the drugs given to each patient while assessing drug pharmacokinetics and biodistribution, as well as tumor response, all in a noninvasive and real-time manner. As a result, physicians will be able to avoid the prolonged use of nonresponsive therapies to treat cancer; instead, they will be able to alter or design innovative treatment regimens, tailored to each individual case, to improve overall survival.
Due to the continued advances in nanotechnology, including design and synthesis of new nanocarriers, the field of theranostics has evolved so that now it is directed more toward using multifunctional nanosystems, which simultaneously deliver both therapeutic and imaging moieties. Nanoparticles (NPs) can accommodate imaging probes, therapeutic agents, and/or targeting agents. This “all-in-one” approach allows assessment of both the pharmacokinetics and pharmacodynamics of the therapies. Figure 18.1 depicts the concept of theranostics based on NP platforms combining the therapeutic and diagnostic functions into one package.
The chapter will focus on the use of theranostics in cancer imaging and therapy. Medical imaging modalities are first introduced, followed by an overview of commonly used nanocarriers for drug delivery. A selection of multifunctional nanoplatforms for theranostic applications are then presented in detail.
18.2 MEDICAL IMAGING MODALITIES
Various types of medical imaging procedures allow the evaluation of organ functions and assist in the diagnosis of disease. Many of these imaging procedures are now being investigated for their application to theranostics.
Magnetic resonance imaging (MRI) produces images by measuring the radio-frequency signals that are given off by magnetized protons in living tissue. Image contrast of different tissues is created because protons in each tissue possess their own characteristic magnetic properties. Image contrast also can be enhanced with the aid of contrast agents that are delivered into the tumor tissue. Contrast agents are paramagnetic materials that can alter the magnetic properties of water protons to enhance the signal in the tissue of interest and help delineate it from that of the normal surrounding tissue. MRI is a beneficial imaging technique because it offers high spatial resolution without any tissue-penetrating limitations. Currently, highly stable gadolinium (Gd) complexes are commonly used to produce bright signal enhancement, while paramagnetic iron oxide nanoparticles (IONPs) generate dark signal enhancement.
Computed tomography (CT) is one of the most commonly used modalities in diagnostic imaging–based x-ray attenuation. CT provides superior visualization of structures with high densities but is limited when used to distinguish soft tissues that have similar densities. CT contrast agents are introduced in order to improve image contrast of soft tissue structures with similar or identical densities. CT contrast agents are composed of biocompatible materials containing elements of high atomic numbers. Clinical CT contrast agents are generally iodinated compounds. NPs containing high-atomic-number elements, such as gold, are now being investigated as CT contrast agents.
Positron emission tomography (PET) and single-photon emission computed tomography (SPECT) are nuclear imaging techniques used to map physiological and biological processes in the body following the administration of radiolabeled tracers. In PET, positrons (positively charged electrons) emitted from the nucleus during decay travel a few millimeters in the tissue, where they undergo annihilation by colliding with electrons. Each annihilation event releases two gamma-ray photons of equal energy (511 keV) in opposite trajectories (180° apart). PET scanners utilize the simultaneous detection of these two photons to precisely locate the source of the annihilation event. Positronemitting isotopes 15O, 13N, 11C, 18F, 64Cu, 62Cu, and 68Ga are often incorporated into biorelevant materials to follow their distribution and concentration, and to detect malignant diseases. SPECT employs gamma-emitting isotopes, such as 99mTc, 111In, and 123I, as probes to detect biomarkers and to label NPs. SPECT imaging can quantitatively determine disease-related biomarkers for diagnostic imaging, to track biodistribution of NPs and to assess therapeutic efficacy.
Optical imaging utilizes photons of a characteristic wavelength to excite fluorescence materials, to visualize biomarkers and to study the localization of materials. Optical imaging offers a high sensitivity and the capability of multicolor imaging. A major limitation of optical imaging is the small depth of light penetration, ranging from hundreds of microns to several centimeters. Near-infrared (NIR) imaging has proven to be more desirable owing to better tissue penetration and less interference from tissue autofluorescence.
18.3 NANOCARRIERS FOR THERANOSTICS
Nanocarriers for theranostics may be constructed from a wide range of organic and inorganic materials, including lipid-based systems (including liposomes, polymersomes, polymeric micelles), polymeric NPs, gold NPs, iron oxide NPs, quantum dots (QDs), silica NPs, calcium phosphate NPs, and various types of carbon nanomaterials (including carbon nanotubes [CNTs]). These carriers have been widely investigated as carriers for drug targeting and delivery and, as such, are described in detail in Chapter 5. The drug carriers described in this chapter can be seen as an extension of this research, whereby the drug delivery system incorporates not only a drug but also imaging agents (contrast agents, fluorescent probes, and radiolabels), for use in diagnostic imaging. Some of these nanocarriers also are cytotoxic via magnetic hyperthermia and photothermal ablation, as well as photodynamic therapy (PDT).
A particular advantage that NPs offer for theranostic purposes is that they can be targeted to specific disease sites, e.g., to the tumor/tumor cells, thereby reducing unwanted side effects. Nanocarrier targeting is described extensively in Chapter 5; to summarize here, targeting can be achieved by either (1) passive or (2) active means. Passive targeting exploits the natural distribution profile of an NP in vivo. Due to their size, shape, charge, and other physicochemical characteristics, NPs tend to be recognized as “foreign” by the immune system and are taken up by the reticuloendothelial system (RES). Their accumulation in RES organs such as the liver and spleen is desirable if these are the target organs. Alternatively, NPs can be sterically stabilized, by rendering their outer surfaces more hydrophilic. Typically, this involves covalently attaching polyethylene glycol (PEG) chains to the NP surface. The highly hydrated PEG layer reduces opsonization and RES uptake, thereby prolonging NP circulation time in the blood. This “stealth” technology can then allow sufficient time for the nanocarriers to accumulate at a tumor site, via what is termed the “enhanced permeability and retention” (EPR) effect of tumor vasculature (Maeda et al. 1984). Tumor blood supply is associated with irregularly dilated and leaky blood vessels, with large pore sizes. NPs that are smaller than 100 nm (although it should be noted that the precise particle size is not fully clear and is a subject of debate—see, for example, Chapter 5, Section 5.2.1) can thus extravasate into the tumor tissue, by passing through the pores and preferentially accumulating there.
Active targeting is the use of a specific-targeting ligand, in order to improve uptake and sequestration into the tumor. The introduction of targeting ligands can help increase the target-to-background contrast in medical imaging while also improving the local concentration of the therapeutics at the tumor site and reducing its systemic toxicity. A targeting moiety is often covalently attached to the NP surface. Ideally, a ligand is used which targets a protein that is predominately overexpressed on tumor cells, in order to prevent the delivery vehicle from accumulating elsewhere in the body. For example, folic acid is often used as a targeting motif, as the folic acid receptor is known to be overexpressed on cancer cells.
Due to their very high surface-area-to-volume ratios, NPs have high loading capacities. Drugs and imaging agents can be loaded into nanocarriers. Encapsulated within the nanocarriers, these agents are protected from in vivo degradation, allowing controlled drug release. The NP surface can be modified with targeting ligands, drugs, imaging agents, stealth coatings, etc. Furthermore, the use of external stimuli, including temperature, light, and magnetism, can create activatable NPs, which allow for the selective trigger of drug release and image-based mechanisms. Some NPs demonstrate a pH-dependent solubility profile, which can also be used to control drug release.
18.4 INORGANIC-NANOSIZED THERANOSTICS
18.4.1 GOLD NANOPARTICLE–BASED THERANOSTICS
Gold nanoparticles (AuNPs) have emerged as one of the most extensively investigated theranostic platforms for the diagnosis, imaging, monitoring, and treatment of malignant and other diseases. AuNPs possess a variety of advantageous properties that make them very useful as multifunctional theranostic vehicles. AuNPs offer inherent biological compatibility, an important advantage over many other synthetic delivery systems described later. Furthermore, their large surface area means they can serve as highly efficient carriers, capable of high drug and diagnostic loading. Using simple wet-laboratory techniques, gold nanocarriers have been constructed in a variety of shapes and sizes, including spheres, cubes, rods, cages, and wires; all of which can now be prepared with accurate quality control and in large quantity. They can also be used as either the core or the shell, for polymer–metal and metal–metal hybrid NPs.
AuNPs have high atomic number and induce strong x-ray attenuation, making them effective contrast agents for CT imaging. Because AuNPs are visible by CT, they have potential to be noninvasively assessed in pharmacokinetics, biodistribution, and tumor targeting. AuNP-based theranostics are often developed by surface modification with therapeutic agents and other imaging agents. Photosensitizers (PS), dyes, drugs, and targeting ligands can all be attached to the surface of AuNPs either directly, via amine or thiol groups, or indirectly, using a linker molecule such as bovine serum albumin. Other imaging agents, such as Gd chelates for use in MRI, and radioisotopes for nuclear medicine, are attached to AuNPs for multimodal imaging and image-guided therapy.
Encouraging preclinical data is accumulating on the use of AuNPs as theranostic agents. Chen et al. have recently synthesized and characterized folic acid–functionalized, dendrimer-entrapped AuNPs containing the MRI agent Gd, thereby developing a nanoprobe suitable for both CT and MRI methodologies (Chen et al. 2013). The AuNPs were entrapped within the dendrimer interior and the Gd was modified on the dendrimer surfaces. This multifunctional construct specifically targeted folic acid receptor–expressing cancer cells via a receptor-mediated pathway. Both in vitro cell imaging and in vivo tumor imaging demonstrated significantly improved contrast-to-noise ratios, indicating the potential of this system to detect cancer cells in the body using dual-mode CT/MRI.
AuNPs can undergo surface plasmon resonance. This is an optical phenomenon that arises from the interaction between an electromagnetic wave and the conduction of electrons in a metal. When excited with light at, or near, the absorption maximum (typically in the visible, or NIR, range), the electrons in AuNPs collectively absorb the incoming irradiation and are excited from the ground state to a higher energy level, where they oscillate at a particular resonance frequency. The nonradiative energy relaxation of the electrons that back down to their ground state results in an increase in kinetic energy and ultimately leads to the generation of intense heat into the local environment. Thus, AuNPs can act as energy transducers, converting the absorbed light into heat (see also Chapter 14, Figure 14.18). This photothermal effect can be harnessed for cancer therapy: AuNPs accumulate at a tumor site and are subsequently illuminated; the heat spike can then selectively damage the tumor tissue, while normal tissue is unharmed. In this application, the AuNPs are not functioning as carriers for a cytotoxic drug; rather the AuNPs themselves are causing tumor cell death, via hyperthermia.
Spherical AuNPs, with a characteristic absorption at 500–600 nm, are not appropriate materials for such an application. In contrast, if the morphology is changed to a hollow AuNP construct, such as a nanocage or nanoshell, this can shift the absorption to the NIR region, between 600 and 1000 nm. This is the therapeutic window whereby the interaction of light with biological tissues is low, keeping attenuation and scattering effects to a minimum, reducing unwanted interactions with the surrounding healthy tissue.
Reports on the success of hollow gold nanospheres (HAuNS) for photothermal ablation are increasing. For example, Melancon et al. conjugated the targeting ligand C225 (an antibody directed at epidermal growth factor receptor) to the surface of HAuNS and then evaluated their distribution in mice (Melancon et al. 2008). It was shown that C225-HAuNS had a significantly higher uptake in the tumors, compared with IgG-conjugated HAuNS controls. The efficacy of photothermal ablation was also assessed. Magnetic resonance thermal imaging revealed that for mice injected with C225-HAuNS, the exposure to low doses of NIR light resulted in average maximum temperatures of 65°C, whereas the saline control mice resulted in an average maximum temperature of only 47° after laser treatment. Furthermore, histological analysis showed that tumors treated with the C225-coated HAuNS developed significantly larger necrotic areas than the control tumors following exposure to the NIR laser.
Gold nanorods (GNRs) have also demonstrated potential for photothermal ablation, due to their high light absorption coefficient in the NIR region, good photothermal stability during laser illumination, and high heat generation. GNRs bearing a folate ligand for cancer cell targeting were also labeled with radioactive iodine, in order to monitor NP distribution in vivo during the treatment period (Jang et al. 2012).
18.4.2 IRON OXIDE NANOPARTICLE–BASED THERANOSTICS
IONPs are made from magnetite (Fe3O4). IONPs less than 20 nm are superparamagnetic (i.e., the particles show zero magnetism in the absence of an external magnetic field but can become magnetized in the presence of one) and can be used as contrast agents in MRI. Unlike Gd-based contrast agents, superparamagnetic iron oxide nanoparticles (SPIONs) decrease MR signals of surrounding water protons, resulting in dark signal enhancement. SPIONs are extensively investigated for theranostic purposes. SPIONs need to be surface-engineered to improve their solubility, stability, and performance in vivo. SPIONs are typically coated with hydrophilic materials. A wide variety of coating materials have been used for SPION modification, including dextrans, dendrimers, and polyvinylpyrrolidone. Therapeutics and targeting agents have been covalently conjugated to SPIONs. Biodegradable spacers, e.g., peptides, have been used to facilitate drug release in response to the environment, e.g., within lysosomes. This ensures release of the drug into the cytosol of cells, upon reaching the acidic endosome/lysosome compartments after cellular uptake (Kohler et al. 2005). Small-sized SPIONs are often incorporated into other larger drug carriers, including liposomes, polymersome, polymeric NPs, and silica NPs, to enable visualization of the larger carriers noninvasively with MRI.
Similar to the photothermal ablation described in Section 18.4.1
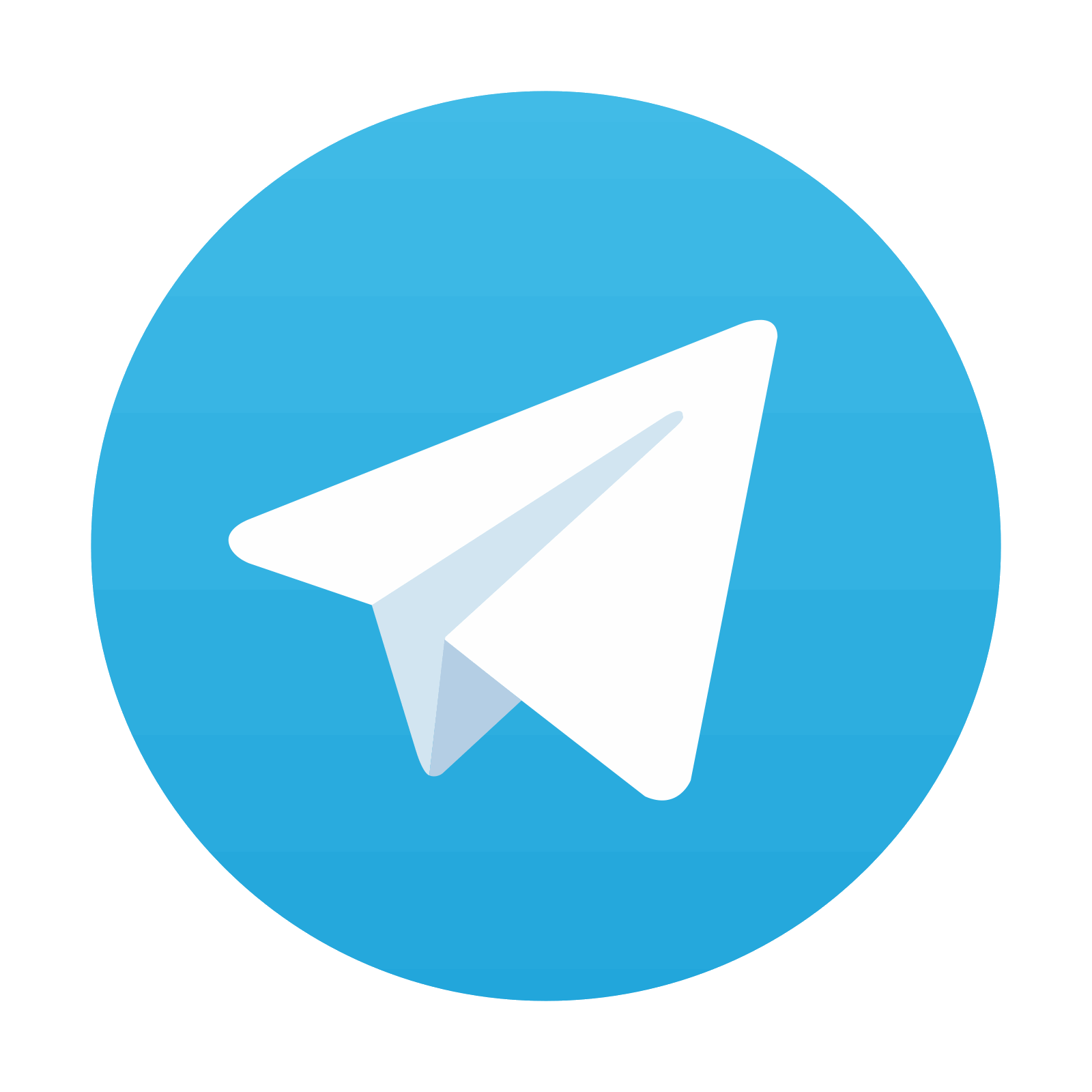
Stay updated, free articles. Join our Telegram channel
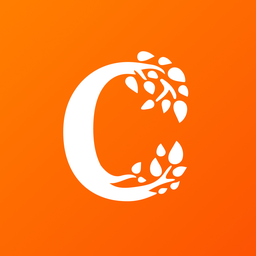
Full access? Get Clinical Tree
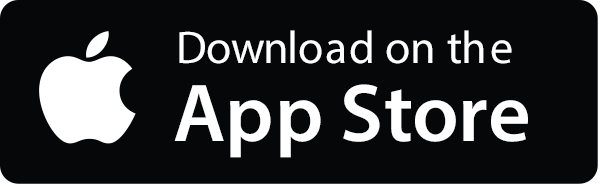
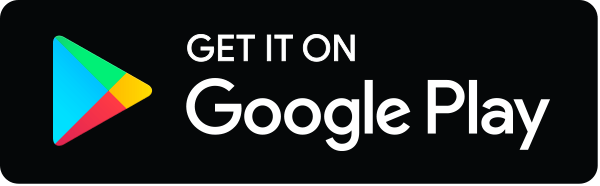