Fig. 6.1
Principle variables and interactions in pulmonary administration of medicines
For many inhalation devices, the inhalation manoeuvre has an influence on the aerosol generation process. Either the air stream through the aerosolisation device delivers the energy for the aerosolisation process (e.g. for passive dry powder inhalers), or it alters the aerosol properties from the device (e.g. by coalescence or evaporation of droplets from nebulisers and metered-dose inhalers). The aerosol generation device may also influence the inspiratory flow manoeuvre by its resistance to airflow. A high resistance limits the flow rate to be achieved and this influences the deposition pattern in the respiratory tract in a positive way. Generally, a poor understanding exists regarding the precise role of airflow resistance, flow rate and aerosol properties in pulmonary therapies particularly with dry powder inhalers. Therefore, it is the aim of this chapter not only to explain the influence of the inhalation manoeuvre on the working principles of various aerosol generation devices and the mechanisms that govern aerosol deposition in the respiratory tract, but also to unravel some persistent misconceptions.
Administration devices for medicines used to treat asthma and COPD are prescription products, with an exception for some nebulised (medicine) formulations. Medicines such as amphotericin B or antibiotics (colistimethate sodium, tobramycin sulphate or gentamicin) for nebulisation in CF therapy are sometimes still partly prepared by hospital pharmacists, and so are nebulised solutions for bronchial challenge testing. Although product formulation and the method of preparation of formulations for inhalation are not the main subjects of this chapter, recommendations are given in the subparagraphs about nebulisation.
6.2 Definitions and Terms
6.2.1 Definitions in the European Pharmacopoeia: Preparations for Inhalation
The European Pharmacopoeia (Ph. Eur.) describes preparations for inhalation as liquid or solid preparations intended for administration as vapours or aerosols to the lung to obtain a local or systemic effect. These preparations may contain one or more active substances and, depending on the type, also propellants, co-solvents, diluents, antimicrobial preservatives, and solubilising and stabilising agents that do not adversely affect the functions of the mucosa of the respiratory tract or its cilia. The Ph. Eur. refers to three types of administration devices for inhalation preparations: nebulisers, pressured Metered-Dose Inhalers (MDI) and Dry Powder Inhalers (DPI). An appropriate size distribution has to be delivered to the patient so that a significant fraction is deposited in the lung and fine particle characteristics are determined by one of the methods for “Aerodynamic assessment of fine particles” in the Ph. Eur. The Ph. Eur. distinguishes between “Liquid preparations for inhalation” and “Powders for inhalation”.
The liquid preparations for inhalation are divided into (A) preparations intended to be converted into vapour, (B) liquid preparations for nebulisation and (C) pressurised metered-dose preparations for inhalation. They are added to hot water to obtain inhalable vapours or converted into aerosols by continuously operating nebulisers or metered-dose nebulisers, and they can be solutions, suspensions or emulsions. They may be prepared by dilution of concentrated preparations or by dissolution of powders. The pH of liquid preparations for use in continuously operating nebulisers has to be within the range between 3 and 8.5. Suspensions or emulsions have to be readily dispersible on shaking and remain sufficiently stable to enable the correct dose to be delivered.
Powders for inhalation are not defined other than that they are presented as single-dose or multidose powders and that the active substances may be combined with a suitable carrier to facilitate their use. If the powder is for a single-dose (pre-metered) inhaler, the device is loaded with powders pre-dispensed in capsules or other suitable pharmaceutical forms. For inhalers using a powder reservoir, individual doses are isolated from the bulk with a metering mechanism within the inhaler.
Important for all different preparations and delivery devices is that they meet the requirements for uniformity of the delivered dose and the number of deliveries per inhaler for multidose inhalers. Also the fine particle dose has to be tested and calculated, but there are no specifications in the Ph. Eur. to meet this most important parameter. Some specific terms and definitions related to pulmonary administration of medicines are given and explained in this paragraph. For various terms different definitions or explanations are given in the literature and specific product information leaflets and it is important to recognise the implications of that.
6.2.2 The Other Definitions
6.2.2.1 Adhesive Mixture
An adhesive mixture is a type of formulation for micronised active substances for inhalation in which the small active substance particles adhere by natural forces (mainly Van der Waals forces) to the surface of much larger carrier (or host) particles.
6.2.2.2 Aerodynamic Diameter (DA)
The aerodynamic behaviour of aerosol particles depends on their diameter, density and shape. To compare the behaviour of particles that have different properties with each other, the aerodynamic diameter (DA) has been introduced, which standardises for particle shape and density. By definition the aerodynamic diameter of a particle is the diameter of a sphere with unit density having the same terminal settling velocity as the particle in consideration. Only for aqueous droplets with a spherical shape and unit density the aerodynamic diameter equals the geometric diameter. For non-spherical particles, the aerodynamic diameter can be expressed in terms of equivalent volume diameter (DE), particle shape factor (χ) and particle density (ρ) (see definitions): DA = DE.(ρ/χ)0.5
6.2.2.3 Aerosol
An aerosol is a (colloidal) dispersion of particles in a gas, which for therapeutic aerosols is air. There is no definition for the particle size distribution of an aerosol, but most airborne particles are within the size range between 0.2 and 20 μm.
6.2.2.4 Breathhold Pause
This is the period during which breathing is interrupted after inhalation of an aerosol. By not immediately exhaling after inhalation, particles in the central and peripheral airways are given time to deposit by sedimentation and make contact with the epithelial lining fluid of the airways.
6.2.2.5 Carrier Particle
See adhesive mixture. Carrier particles in marketed formulations are exclusively alpha lactose monohydrate crystals, mainly in size distributions between 20 and 150 μm, but they may contain substantial amounts of fine lactose < 10 μm.
6.2.2.6 Deposition
This is the act of bringing an aerosol particle in contact with the airway wall. Different deposition mechanisms exist and it depends primarily on the particle’s aerodynamic diameter (see definition) and velocity in which part of the airways an aerosol particle is most likely to be deposited.
6.2.2.7 Deposition Mechanism
Deposition mechanisms are principles by which particles can be deposited onto airway walls. For inhaled aerosol particles only two major mechanisms are important: inertial deposition and sedimentation (see definitions). In the literature also diffusion, interception and electrostatic precipitation are sometimes mentioned as deposition mechanisms, but these mechanisms, if occurring at all, are of lower relevance.
6.2.2.8 Deposition Modelling
Deposition modelling consists of simulation of the deposition in the lungs on the basis of deposition probability equations for inertial impaction, sedimentation, and diffusion.
6.2.2.9 Dose
The dose is the amount of active substance (to be) delivered from the inhalation device. Different definitions for the dose can be given for MDIs and DPIs. Basically, there is a difference between the label claim (also: nominal dose) and the delivered dose (also: emitted dose). The label claim is the dose as measured into the dose compartment of the device (for single-dose or multiple unit dose dry powder inhalers) or as measured by the device (for MDIs and multidose DPIs which both have a metering chamber). The delivered dose is the amount of active substance leaving the mouthpiece of the inhaler, which is lower than the label claim due to inhaler (mainly in the mouthpiece) retentions (for MDIs and DPIs) and incomplete emptying of the dose compartment (for DPIs only). Some manufacturers of DPIs weigh between 10 % and 20 % more than the label claim into the dose compartments to compensate for the inhaler retention, whereas others use an average delivered dose as label claim. This shades the difference between label claim and delivered dose. Delivered doses vary not only between devices; for DPIs they mostly also depend on the flow rate for the same type of device. A special situation concerns nebulisers where the delivered lung dose may not only depend on the retention in the nebulisation cup, but also on aerosol losses during periods of exhalation. Next to (nominal or delivered) dose, the fine particle dose (or fraction) is important (see FPD and FPF).
6.2.2.10 Drag Force (FD)
Particles moving relative to the surrounding air are subjected to a resisting force by collision with air molecules. This force is the same whether the particle moves through the air or the airflows past the particle. For small airborne aerosol particles the resisting force, or drag force (FD), is described by Stokes’ law: FD = 3.π.η.U.D, to which several correction factors may be applied (as for the shape factor: see definition). In this equation η is the dynamic viscosity of the air, U is the particle velocity (relative to the air) and D is the particle diameter.
6.2.2.11 Equivalent Volume Diameter (DE)
The equivalent volume diameter (DE) of an irregularly shaped particle is the diameter of a sphere having the same volume as the particle in consideration. The equivalent volume diameter is used to describe the dynamic particle behaviour of non-spherical particles in combination with the shape factor (see definition).
6.2.2.12 Fine Particle Dose (FPD) and Fine Particle Fraction (FPF)
Fine particle dose (FPD) and fine particle fraction (FPF) have to be defined by particle size (distribution). Based on their ability to target the site of action in the lungs, FPDs in the literature are frequently defined as the mass fractions of particles < 5 μm in the delivered aerosol. However, particles < 1 μm are not desired as they are exhaled to large extent, whereas for total and deep lung deposition particles in the narrow size range from 1 to 3 μm may be more appropriate. FPD is given in microgram or milligram active substance. FPF is a relative measure of FPD, expressed as percent of the dose for which both the label claim and the delivered dose can be used (see label claim).
6.2.2.13 Geometric Standard Deviation (GSD)
Geometric standard deviation (GSD) is a measure of the distribution of particle sizes which can be used for log-normal volume (or mass) distributions as function of the diameter:
D15.87 is the diameter corresponding with 15.87 % cumulative volume (or mass) and D84.13 is the diameter corresponding with 84.13 % cumulative volume (or mass).
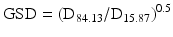
6.2.2.14 Impaction Parameter (IP)
The impaction parameter (IP) of an aerosol particle is the product of the particle density (ρ), the square of the particle’s (aerodynamic) diameter (D) and its velocity (U):
The parameter predicts the chance of impaction against an obstruction in the flow direction of the particle and can for instance be used to predict oropharyngeal deposition. Practically, instead of particle velocity sometimes the flow rate (Φ) through an inhaler is used, but this does not enable comparative evaluations between different inhalers when the cross sections for airflow in the mouthpieces are different between the inhalers as this will result in different velocities.

6.2.2.15 Impactor
Multistage impactors or cascade impactors are used for aerosol particle size analysis. By drawing a constant flow rate through an impactor nozzle, airborne particles may or may not be collected on an impaction plate underneath the nozzle depending on their aerodynamic diameter and velocity. The cut-off diameter of an impactor varies with the flow rate through the nozzle, and by placing impactors with decreasing nozzle diameters in serial arrangement a mass distribution as function of the aerodynamic diameter can be obtained. The United States and European Pharmacopoeias show different types of impactors to be used for aerosols of which the nine-stage Andersen impactor is most popular in the USA and the seven-stage Next Generation Impactor (NGI) is most frequently used in Europe.
6.2.2.16 Inertial Impaction
One of the two dominant deposition mechanisms for aerosol particles in the respiratory tract (see definitions) is inertial deposition or impaction. Inertial deposition is based on the particle’s inertia or momentum, which is the product of particle mass (m) and velocity (U). Inertial deposition occurs particularly in the upper respiratory tract where air velocity is high and the largest aerosol particles are still airborne.
6.2.2.17 Inspiratory Flow Rate
The volume of air per unit time through an inhaler during inspiration is the inspiratory flow rate, which is expressed in L/min. Generally, the flow rate through an inhaler quite rapidly reaches a maximum value (peak inspiratory flow, PIF) followed by a slower decrease to zero flow. As a general rule, the average flow rate equals approximately 70 % of PIF. From the average flow rate and the total inhalation time the inhaled volume (V) can be computed. The flow rate influences the particle size distribution in the aerosol and the deposition pattern in the respiratory tract.
6.2.2.18 Label Claim
The label claim of DPIs and MDIs is the amount of active substance corresponding with a unit dose. Different label claims are used and there is a tendency in Europe to change from metered dose to delivered dose for the label claim, which is an estimated value for the amount of active substance leaving the mouthpiece. For fine particle fractions (FPFs) it is important to know which type of label claim has been used as reference, or a comparative evaluation between different devices will be impossible.
6.2.2.19 Median Diameter
The median diameter corresponds with the 50 % value of a cumulative number, volume or mass percent distribution as function of the diameter. Fifty percent of the volume (number or mass) of the aerosol is in larger, and 50 % is in smaller particles than the median diameter. For a volume distribution it is the volume median diameter, for a mass distribution the mass median diameter. When the mass percent is expressed as a function of the aerodynamic diameter, reference can be made to the mass median aerodynamic diameter (MMAD).
6.2.2.20 Mass Median Aerodynamic Diameter (MMAD)
The MMAD is a parameter frequently used to characterise therapeutic aerosols. MMAD alone is not very useful however, as it provides no information about the size distribution in the aerosol and the mass fraction of the dose (label claim) processed into a suitable aerosol. Fine particle dose and fraction are more meaningful parameters, particularly for DPIs (see definitions).
6.2.2.21 Monodisperse Aerosol
In a monodisperse aerosol all particles have the same diameter. In practice, monodisperse aerosols are very difficult to obtain and therefore, aerosols are considered monodisperse when their geometric standard deviation (GSD) is smaller than 1.2 (see definition).
6.2.2.22 Plume Velocity
The plume velocity is the velocity with which an aerosol is released from an MDI. Generally, the plume velocity from hydrofluoroalkane (HFA) holding MDIs is much lower than that from chlorofluorocarbon (CFC) holding MDIs, but this may depend on the presence of a co-solvent.
6.2.2.23 Polydisperse Aerosol
In a polydisperse aerosol the particles have different diameters and the size distribution is such that the geometric standard deviation (GSD) has a value larger than 1.2 (see definitions).
6.2.2.24 Resistance (Against Airflow)
Inhalers are flow constrictors which reduce the flow rate (Φ) to be achieved during inhalation. Their behaviour in this respect complies with the general equation for orifice types of airflow resistances (Φ = Fu(A).√dP), where Fu(A) is a function of the cross section (A) for airflow and dP is the pressure drop across the inhaler (in kPa). Fu(A) may be complex but fairly constant over a wide range of flow rates and contains flow coefficients depending on the precise inhaler design. The reciprocal value of Fu(A) is the inhaler’s resistance to airflow (R).
6.2.2.25 Sedimentation
One of the two dominant deposition mechanisms for aerosol particles in the respiratory tract (see definitions) is sedimentation or stationary settling. Sedimentation occurs under influence of the force of gravity and settling (falling) particles reach a terminal (stationary) settling velocity once the force of gravity is in equilibrium with the drag force (see definitions). The terminal settling velocity (UTS) is proportional to the square of the particle diameter (D) and also depends on particle density (ρ) and shape factor (χ: see definitions):
UTS = (ρ.D2.g.Cc)/(18η.χ) in which g is the acceleration of gravity, Cc is the Cunningham correction factor for slip flow and η is the dynamic viscosity of the air.
6.2.2.26 Shape and Shape Factor (χ)
Only aqueous aerosol droplets and some particles obtained from (spray) drying of droplets are perfectly round. Most solid aerosol particles have other shapes, and also when spherical particles cluster together their shape changes from round into irregular. The shape of a particle affects its drag force (see definition) and in particle dynamics shape is characterised by a (dynamic) shape factor. This factor for a non-spherical particle is defined as the ratio of the actual resistance force to the resistance force of a sphere having the same volume and velocity relative to the air. The factor is applied to make corrections for Stokes’ law for the drag force (see drag force) which influences both inertial impaction and sedimentation.
6.2.2.27 Stopping Distance
The stopping distance or inertial range is the distance a particle will travel in still air with all external forces eliminated, except for the drag (resistance) force of the air which decelerates the particle to zero velocity. The stopping distance depends on the particle’s momentum, which is the product of particle mass and velocity (m.U).
6.2.2.28 Target Area
The target area is the area in the respiratory tract where the action of the inhaled medicine is most needed. The target area may either be part of the respiratory tract or the whole lung, depending on the medicine.
6.3 Biopharmaceutics
Aerosol particles carrying the active substance have to make contact with the walls of the respiratory tract in the target area and the medicine has to be dissolved before it can become active. In this paragraph, the anatomy of the human respiratory tract is described in view of its function as transport route and target area for inhaled medicines.
6.3.1 The Human Respiratory Tract
For pulmonary administration of medicines the mouth is the port of entry (see Fig. 6.2). Inhaled air carries the aerosol from the inhalation devices past the oral cavity, pharynx and larynx before it enters the tracheobronchial tree. Starting with the trachea (generation 0) most airways branch into two (some in more) smaller airways, which comprise the following generation. The trachea branches (bifurcates) into two main bronchi (generation 1) which bifurcate further into five lobar bronchi (generation 2) and so on, until eventually the alveolar sacs (alveoli) are formed. Estimates for the number of branchings (airway generations) from the trachea to the alveoli in the literature vary between 20 and 28 and the number of airway ducts in each generation is roughly 2 to the power of the generation number; for example, in generation 8 there exist 28 (256) airway ducts. The number of alveoli does not comply with this geometric sequence however, and is much higher up to an estimated 300–800 million [1]. The airway system can be subdivided in many different ways. Clinically, large airways (with diameters > 2 mm) are frequently distinguished from small airways (with diameters < 2 mm). In addition, the terms upper and lower airways are frequently used, but with different definitions. From the viewpoint of fluid and particle dynamics dividing the airways into conducting, transitional and peripheral airways seems more practical. Clear definitions for these regions have never been given but on the basis of airflow velocity and functional and anatomical differences the conducting airways are referred to as the generations 0–11, the transitional airways as the generations 12–16 and the peripheral airways as the generations 17–23 in this chapter. In aerosol deposition studies with radiolabeled substances partitioning is often into central, intermediate and regional airways on the basis of two-dimensional γ-camera images. The average angle of bifurcation is 37° which from the fluid dynamics point of view is the angle with the least disturbance of the flow pattern. Lung models used for deposition simulation in the literature mostly give only 23 generations (e.g. the Weibel model) and only few are extended to 26 generations (e.g. the Hansen and Ampaya model) [2].
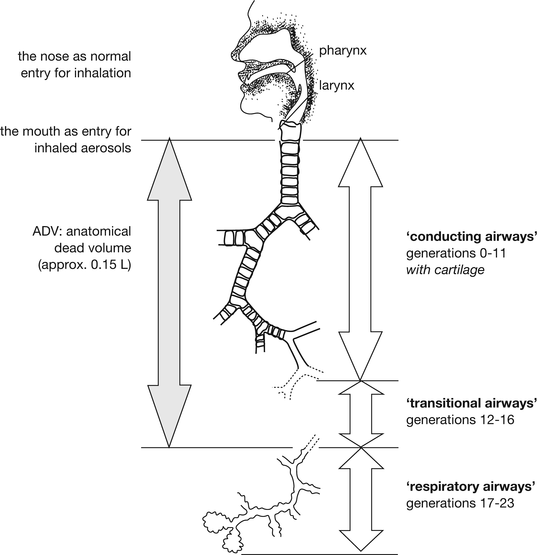
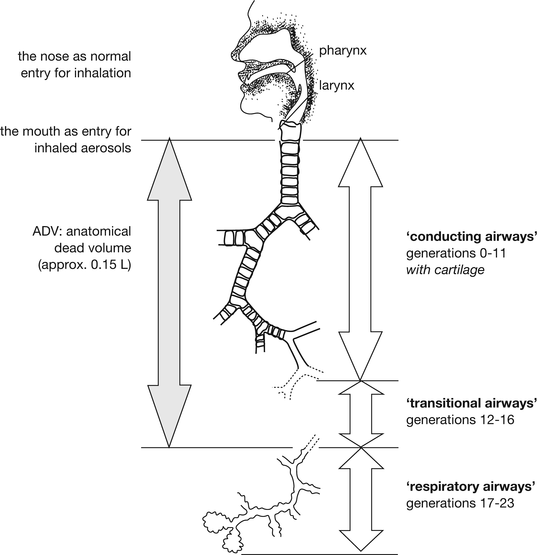
Fig. 6.2
The airways as transport route for aerosols. The anatomical dead volume of 0.15 L is for adults; the total number of airway generations (23), based on the Weibel model, is by approximation. Source: Recepteerkunde 2009, ©KNMP
From the trachea to the small bronchi cartilage is present in the walls of the airways. In the trachea cartilages are C-shaped, in the bronchi they appear as interspersed small plates of elastic tissue. The cartilages in the trachea are joined by smooth muscle which continues into the bronchi and bronchioles where the muscles encircle the airways completely. Further down the respiratory tract, smooth muscle becomes less until it is absent in the alveoli. The airways are covered with epithelium of which the type varies within the tract. There are glands (upper respiratory tract) and mucus producing goblet cells, and most of the epithelial cells (into the bronchi) are ciliated cells. The cilia beat upwards, moving mucus (including all, in the mucus, entrapped foreign inhaled particles) towards the throat where it is swallowed. In the alveoli neither mucus nor ciliated cells are present and mainly alveolar macrophages are responsible for destroying foreign material. The walls of the alveoli contain surfactant secreting cells. Surfactant decreases the surface tension of the alveolar lining fluid preventing collapse and assisting re-inflation of the lung after exhalation.
Different lung models are used to describe the human airways as transport route for inhaled aerosol particles [2]. All these models have in common that they are simplifications of reality presenting the airways as round pipes with defined lengths and diameters. From the trachea with an estimated diameter of 15–18 mm (in adults) the airway diameter decreases towards the alveolar ducts, whereas the number of airways increases. The increase in number (from 1 to approximately 8 × 106 , assuming 23 generations) is much higher than the decrease in diameter (from 18 to approximately 0.4 mm). As a consequence, the cross section for airflow, after an initial decrease in the first four generations, increases exponentially towards the alveoli, which corresponds to an exponential decrease of the air velocity. In the terminal bronchioles the air stands practically still and its velocity reaches the same value as the terminal settling velocity of particles in the size range between 5 and 6 μm.
6.3.2 Spirogram and Lung Volumes
The total lung capacity (TLC) of healthy adult subjects varies between approximately 4 L (female) and 6 L (male), but during tidal breathing at rest only a fraction of this volume is refreshed: approximately 0.5 L. During severe exercise, this tidal volume (TV) increases to about 1.5–2.5 L, which means that a considerable part of the TLC is not used. In fact, a residual volume (RV = approximately 25 % of TLC) cannot be exhaled at all to prevent collapse of the lungs. Tidal breathing is not on top of the residual volume, but on top of the functional residual capacity (FRC), which after exhalation leaves a volume of about 2.3 L of air in the lungs for an adult male. The alveolar volume is about 2.1 L and this implies that during tidal breathing air refreshment by convective transport takes place mainly above the alveolar volume. Hence, to reach the alveoli effectively with an inhaled aerosol, a preceding exhalation to residual volume is necessary.
6.3.3 Target Areas for Inhaled Medicines
The precise area to target in the lungs depends on the type of medicine given and the mechanism of action for this medicine. Most of the active substances used for inhalation interact with cell receptors in the respiratory tract [3]. For asthma and COPD these include mainly β2-adrenoceptor agonists and muscarinic receptor antagonists (anticholinergics). The distribution of receptors over the lung is an important determinant of both the clinical effect of the medicine and the desired site of deposition for the active substance. The primary action of β2-agonists is to relax airway smooth muscle. β2-agonists target β2-receptors that are present in high concentration in lung tissue and localised to several cell types which, next to smooth muscle, are epithelium, vascular smooth muscle and submucosal glands [3]. They also target β1-receptors localised to submucosal glands. There is a uniform distribution of β-receptors also on the alveolar wall with a ratio of β1 to β2 receptors of 2:1. The density of β2-receptors in airway smooth muscle does not change down the respiratory tract and is the same in small and large airways. Therefore β2-agonists may dilate all airways and this is relevant to asthma and COPD where small airways are involved. To achieve acute relief of bronchoconstriction, reaching the larger airways, which have the highest resistance to airflow, is mostly sufficient.
Inhaled anticholinergics are the most effective class of bronchodilators in COPD patients. Muscarinic receptors are localised to smooth muscle of all airways, but the density decreases down the respiratory tract. They are also localised to airway epithelium and submucosal glands [3]. Four subtypes of muscarinic receptors exist in the lungs and those mediating bronchoconstriction belong to the M3-receptor subtype on endothelial cells which release nitric oxide (NO). Muscarinic receptors of the M3 subtype also mediate mucus secretion and so do receptors of the M1 subtype, whereas autoreceptors in the human airways belong to the M2 subtype. Medicines like ipratropium bromide block prejunctional M2-receptors and postjunctional M3-receptors in airway smooth muscle with equal efficacy. The presence of M2-receptors has also been demonstrated in airway smooth muscle and M1– and M3-receptors are both present in submucosal glands, whereas M1-receptors can also be found in the lung parenchyma. Recently, it has been suggested that muscarinic receptors may have a much greater role in the pathophysiology of obstructive airway diseases than previously thought [4]. Active substances like tiotropium may potentially inhibit airway inflammation and remodelling, and it has recently been shown that aclidinium may play an important role in inhibiting fibroblast-myofibroblast transition, which is a key step in peribronchiolar fibrosis formation [5]. Deposition in the whole lung is therefore desirable for anticholinergics in spite of the fact that cholinergic activity in the lung is most pronounced in the large airways [6].
Inhaled corticosteroids (ICSs) are the mainstay of asthma management. Their effects are mediated by glucocorticoid receptors in target cells of the lung. Almost every cell has glucocorticoid receptors, but the number per cell varies with the type of tissue and in the airways the highest density is found in endothelial and epithelial cells [3]. Airway epithelial cells, which express multiple inflammatory proteins dominating the inflammation in asthma, may be the major target for ICSs. Because epithelial cells are present throughout the entire lung, all airways have to be targeted with ICSs.
Many specific mediator receptors are involved in asthma but they are so abundant that specific antagonists for these receptors have little effect, with an exception for cysteinyl leukotriene-1 (cys-LT1) receptors which are distributed predominantly on airway smooth muscle and (to a lesser extent) on macrophages. Their numbers are small, however, and this may explain why antileukotrienes like montelukast and zafirlukast, which prevent predominantly leukotriene-induced bronchoconstriction, are less effective than β2-agonists.
6.3.4 Side Effects and Toxicity
Side effects can be the result of unwanted systemic action, toxicity, irritation and hypersensitivity following sensitisation. Both the active substances and excipients can cause side effects and in addition to the chemical nature of the inhaled compounds, also physical properties can be relevant. An example can be given for salbutamol, for which it has been shown that increasing the dose may result in increased side effects without improving the therapeutic effect [7]. Also specific salts of an active substance may be less favourable, as they can increase the degree of irritation from particle deposition on the mucosa [8]. Irritation may result in severe cough and chest tightness; both may further depend on the precise site of deposition which depends on particle size and/or flow rate. Dry powder formulations for low dose substances in asthma and COPD therapy previously contained only alpha lactose monohydrate as carrier (or diluent) excipient. In some countries, there has been concern about the use of lactose in inhaled medication because of some rare cases of bovine spongiform encephalopathy (BSE), but it is highly unlikely that the prions causing BSE can be found in this excipient. Currently, many formulations are introduced to the market which contain magnesium stearate as force control agent to improve powder dispersion (e.g. Chiesi Foster NEXThaler®, Novartis Seebri Breezhaler® and GSK Breo Ellipta®). Although the use of this practically insoluble excipient has been approved, its long term safety may be questioned. Still uncertain are also the long term effects of various excipients in high dose medicines, such as lung surfactant (dipalmitoylphosphatidylcholine, DPPC) in various particle engineered powders, hydrogenated soya phosphatidylcholine (HSPC) and cholesterol in liposomal formulations, and poly lactic acid (PLA) and poly lactic-co-glycolic acid (PLGA) in insoluble microspheres. The arguments for safety are that the compounds are not foreign to the lungs or that they do not interfere with physiological processes. However, interference for substances like DPPC are likely to depend also on their concentration, and for instance metabolic lactic acid is an important mediator of myofibroblast differentiation via a pH-dependent activation of transforming growth factor-β [9]. MDI formulations contain various excipients too, of which some have been less abundantly used since the replacement of CFC by HFA propellants. Furthermore, most HFA-MDIs have a lower plume velocity and a higher plume temperature than CFC devices which reduces the cold-freon effect and local side effects from substantial throat deposition.
6.4 Mechanisms of Aerosol Deposition and Aerosol Characterisation
6.4.1 Forces Acting on Inhaled Aerosol Particles
Aerosol particles transported in a steady laminar air stream have basically the same velocity and flow direction as the air, but in contrast with the air molecules particles have a much higher inertia. Therefore, they cannot follow rapid changes in velocity or direction of the airflow, which for instance occur in curved airways, at bifurcations or around local obstructions. In such areas or in turbulent air streams particle trajectories and velocities may differ from the stream lines of the air and under these conditions, a drag or resistance force of the air is added to the force of gravity acting on the particle. Whereas the particle’s inertia tends to maintain particle motion in the original direction, the drag force tends to change this direction into that of the air stream. The tendency of a particle to maintain its state of motion in still air is expressed by its stopping distance (S) which is related to the particle momentum. For the sake of simplicity and an easier understanding, it can be imagined that a particle following a curvilinear trajectory is subjected to a centrifugal force. This adds a third force acting on particles in a bent airway as depicted in Fig. 6.3.
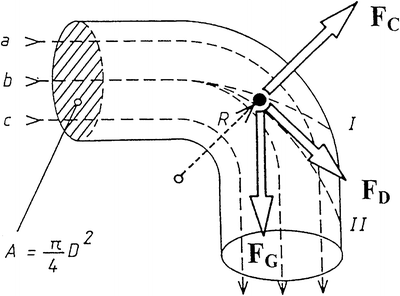
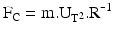

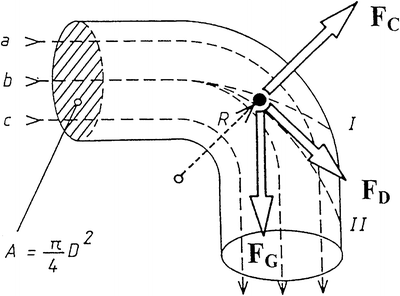
Fig. 6.3
Forces acting on airborne particles in a bent airway. Source: Recepteerkunde 2009, ©KNMP
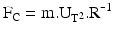
(6.1)

(6.3)
Where:
m is the particle mass
UT is the tangential velocity
R is the radius of the bent
η is the dynamic viscosity of the air
UPA is the particle velocity relative to the air velocity
D is the particle diameter
g is the acceleration of gravity
Of these forces, the centrifugal force and force of gravity are a function of the particle mass, which is proportional to the third power of the particle diameter, whereas the drag force is proportional to the first power of the diameter. The drag force and the centrifugal force depend on the particle velocity, whereas the force of gravity does not. This has the important implication that the balance between these forces can be influenced by changing the particle diameter, particle velocity, or (as a determinant for particle mass) particle density. Because the particle shape influences the drag force, the shape factor is a fourth determinant for a particle’s aerodynamic behaviour.
6.4.2 Deposition Mechanisms in the Respiratory Tract
The balance between the three forces acting on airborne particles results in dominance of either inertial impaction or sedimentation, which are the two main deposition mechanisms in the respiratory tract. Inertial impaction occurs when particles have a high momentum and the air suddenly changes its direction. This situation is met in the human throat and the upper airways where the air velocity is high and the largest particles in the aerosol are still present in the inhaled air stream. For such particles the ratio of centrifugal force to drag force is relatively high, meaning that they have a great chance of colliding with the airway wall based on their high inertia, which event is referred to as inertial impaction. As the largest particles are removed in the upper airways and the air (and thus particle) velocity decreases down the respiratory tract, particle inertia and the drag force decrease and the force of gravity becomes more dominant. This leads to settling (falling) of particles in the central and peripheral airways and when the settling time is long enough it could result in deposition by sedimentation. For a small fraction of the finest particles (D ≪ 1 μm) Brownian motion may become noticeable. This mechanism of displacement, resulting from particle collision with surrounding air molecules, causes particle movement with a randomly changing direction which could lead to contact with the wall of an airway. However, because the displacement velocity by Brownian motion (or diffusion) is very low, and so is the mass fraction of the dose represented by particles smaller than 1 μm, this mechanism of deposition does not contribute substantially to total deposition. Also electrostatic capturing of particles is mentioned in the literature as a possibility to bring aerosol particles in contact with airway walls but for the relevance of this mechanism there is no experimental evidence. One aspect that is still relatively unexplored and needs further investigation is the possibility that particles change their mass during passage through the respiratory tract, e.g. by moisture sorption [10]. Such particles may be inhaled as small (submicrometer) to avoid high deposition fractions in the oropharynx and larger airways and increase in weight in the high humidity within the airway system to enhance sedimentation deposition in the deep lung.
6.4.3 Sedimentation Takes Time
Particle settling in the respiratory tract occurs under the influence of the force of gravity and the drag force. In the stationary situation these forces counterbalance each other and this enables to calculate the stationary or terminal settling velocity (see terms and definitions) which is proportional to the square of the particle diameter. For spherical particles with unit density in the range of diameters between 0.5 and 5 μm, which covers the range of interest for inhalation, the terminal settling velocities are given in Table 6.1.
Table 6.1
Terminal settling velocities in still air of spherical particles with unit density
Particle diameter (μm) | Terminal settling velocity (μm/s) | Average settling timea (s) |
---|---|---|
0.5 | 7.5 | 30 |
1 | 30 | 7.5 |
2 | 120 | 1.9 |
3 | 271 | 0.8 |
4 | 482 | 0.5 |
5 | 753 | 0.3 |
Table 6.1 shows that particles of 1 μm, or smaller, are less favourable for inhalation because their settling time is too low. Even for a total residence time of 7.5 s in the smallest airways, the falling distance of a 1 μm particle is no more than 50 % of the diameter of that airway, if the air stands completely still. In practice, the air velocity remains much higher than the particle’s settling velocity, even in these most distal airways (e.g. 2.5 mm/s in generation 22 at an inspiratory flow rate of 60 L/min), and this can influence sedimentation in a negative or positive way. Moreover, most distal airways are not horizontal ducts and a falling distance of 50 % of the airway diameter does not provide an average 50 % chance for deposition of particles entering the airway randomly distributed over its cross section. As a consequence of all this, the deposition efficiency of very fine particles is low and exponentially decreases with decreasing diameter. This is reflected in the fraction of small particles exhaled again, which is known for particles in the size range between 1.5 and 6 μm [11]. Figure 6.4 shows the relationship between particle diameter and measured exhaled fraction for monodisperse aerosols, and the extrapolation of this relationship towards particles of 1 μm. The trend computed matches very well the relationship which presents the time needed to fall a distance equal to the diameter of a peripheral airway (0.43 mm).
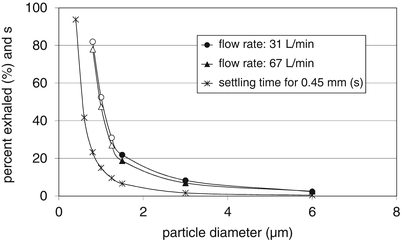
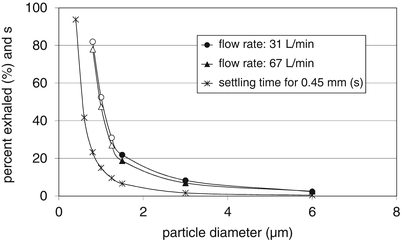
Fig. 6.4
Trends for the percentage particles exhaled and the time needed to fall a distance that equals the diameter of a peripheral airway (0.43 mm), both as function of the aerodynamic particle diameter. The percentage exhaled for 1 μm particles is obtained from extrapolation (using a second order polynomial equation); exhalation data for 1.5; 3 and 6 μm particles derived from Usmani et al. [11] and extrapolation of the correlationships towards particles of 1 μm
6.4.4 The Influence of Particle Shape and Density
The terminal settling velocities and average settling times given in Table 6.1 are for spherical particles with unit density which is the density of water (1 g/cm3). This may well apply for wet aerosol droplets from aqueous solutions of active substances which take a spherical shape as soon as they have been formed under the influence of the surface tension. Solid aerosol particles from dry powder inhalers have different properties. Such particles may exhibit a variety of different shapes and also have different densities, depending on how they were prepared. Particles obtained from micronisation are mostly crystalline and have density values typically in the range between approximately 1.25–1.55 g/cm3. Due to the size reduction process (breaking of larger particles) micronised particles have irregular shapes which, however, never deviate extremely from the spherical shape. This is in contrast to particles obtained with anti-solvent precipitation or super critical drying which can have shapes varying from cubic to plate or needle like. An increasing number of high dose medicines for inhalation are currently produced with spray drying techniques, yielding particles with high internal porosity or corrugated surfaces to enhance dispersion during inhalation. Such particles may largely be spherical, but they have low densities, frequently much smaller than 1 g/cm3. Both the particle density and shape influence the aerodynamic behaviour of aerosol particles and this affects deposition in the human airways. Figure 6.3 shows that the force of gravity and the centrifugal force both depend on the particle density (ρ). All three forces furthermore depend on the particle diameter, but non-spherical particles have different dimensions in different directions and therefore, a unique diameter to characterise such particles cannot simply be given. For this reason, the equivalent volume diameter (DE) was introduced, which is the diameter of a spherical particle with the same volume as the non-spherical particle. Finally, the drag or resistance force strongly depends on the particle shape. This does not show in the equation given in Fig. 6.3, which is therefore only valid for spherical particles. To standardise for both shape and density, the aerodynamic diameter is used, which by definition is the diameter of a sphere with unit density having the same terminal settling velocity as the irregular particle in consideration.
6.4.5 Polydisperse Aerosols and the MMAD
All currently marketed inhaler devices produce polydisperse aerosols of which the individual particles have different sizes. Therefore, they cannot be characterised by a single diameter. In fact, for most solid aerosols from dry powder inhalers the particles may have different shapes too, which is the reason to characterise them with aerodynamic diameters. To be able to express polydisperse aerosols with a single parameter, the median aerodynamic diameter (MAD) was introduced. When the aerodynamic size range which covers the population of particles in the aerosol is divided into different classes and the volume or mass fraction within each size class is expressed as function of the class mean diameter, a volume or mass distribution as function of the aerodynamic diameter is obtained. This volume or mass frequency distribution can be transferred into a cumulative percent distribution of which the 50 % value corresponds with the volume or mass median aerodynamic diameter (VMAD or MMAD). This is the diameter indicating that 50 % of the total aerosol volume or mass is in larger, and 50 % is in smaller particles. When particles of all sizes in the aerosol have the same density, which is mostly the case, then VMAD equals MMAD.
MMAD is frequently presented as the parameter characterising aerosols from inhalation devices best. This is not true however. To judge the quality of a therapeutic aerosol from a particular type of inhaler, more information is needed. The MMAD does not give any information about the size distribution of the aerosol particles. Substantial mass fractions may be outside the desired size range for adequate deposition of active substance in the target area, even when MMAD looks very favourable. Moreover, MMAD does not give information about the mass fraction of the dose (label claim) that has been delivered within the desired size range. For all types of inhalers, the delivered fine particle dose (FPD) is much lower than the label claim and this may vary from 10 % to 60 % for DPIs and up to 90 % for MDIs. For these reasons it is best to define first the FPD in terms of desired size range and mass percent (of the label claim, yielding the fine particle fraction, FPF) and next compute the MMAD for this size range. In the literature, mostly FPF < 5 μm is mentioned as the relevant fine particle fraction, but practically FPF 1–5 μm is more meaningful because of the low deposition efficiency of submicron particles (Fig. 6.4).
6.4.6 Deposition Efficiencies and the Most Preferable Size Distribution
The deposition mechanisms mentioned previously have different efficiencies which, in addition to the particle properties, depend on the velocity with which the particles enter the respiratory tract and their residence time in the tract. The velocity is most important for inertial impaction in the oropharynx and larger (mainly conducting and transitional) airways. The likelihood of particles to be deposited in the mouth and throat or larger airways is a function of their momentum, which is the product of particle mass and velocity and can be predicted with the impaction parameter.
An experimental relationship between impaction parameter (based on inhaled flow rate) and oropharyngeal deposition from a study with monodisperse particles is shown in Fig. 6.5. The highest value in this relationship is for 6 μm particles inhaled at a flow rate of 67 L/min; the lowest for 1.5 μm particles inhaled at 31 L/min [6].
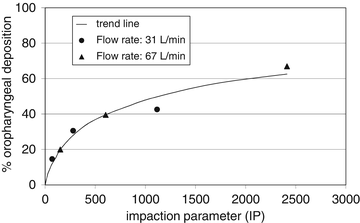
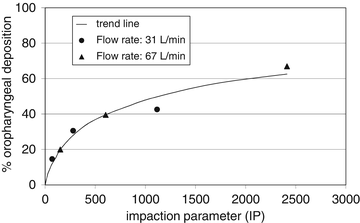
Fig. 6.5
Experimental relationship between percent oropharyngeal deposition and impaction parameter computed as IP = D2.Φ, where D is the aerodynamic particle diameter (micrometre) and Φ is the flow rate with which the particle is inhaled (L/min). Data derived from Usmani et al. [11]
The figure makes clear that substantial lung doses cannot be obtained with particles larger than 6 μm, unless they are inhaled very slowly which from dry powder inhalers is often not possible. Large particles also deposit effectively in the larger airways where initially the velocity increases (until generation 4) before it slows down. This has the consequence that particles larger than 3 μm from DPIs do not effectively enter the peripheral lung.
The residence time is more relevant to small particles which have to deposit mainly by sedimentation in the central and peripheral airways. In these regions, the flow rate is strongly reduced and large particles are not present in large numbers due to which inertial impaction is less prominent. Sedimentation takes time however, as explained above, and particularly for particles in the size range below 1.0–1.5 μm a residence time of several seconds may be needed to obtain a noteworthy deposition by sedimentation. This practically confines the diameter for most effective total lung deposition to the very narrow aerodynamic size range between 1 and 1.5 and 3 μm. Only for bronchodilators which need to target predominantly the larger airways, particles in the size range between 3 and 6 μm may be more effective [11].
6.4.7 Medicine Distribution over the Entire Respiratory Tract
One of the aspects that is often neglected, but may be of utmost importance for effective therapy, is the active substance concentration achieved in different lung regions. Most in vivo deposition studies with radiolabeled substances from dry powder inhalers teach that the deposition fractions in the central, intermediate and peripheral lung are very roughly one third of the total lung dose each [12–14]. This is more or less confirmed by deposition modelling studies with monodisperse particles of 3 μm inhaled at a moderate flow rate of 30 L/min [15], although a good comparison in this respect is not possible as different lung regions are defined differently in these studies. Considering the exponentially increasing internal surface area of the airways from the trachea to the alveoli, which differs roughly by a factor 130 between the generations 0–11 (conducting airways) and 17–23 (peripheral airways) based on the Weibel model, it may be concluded that there must be a dramatic difference in active substance concentration (in μg/cm2) between these regions. A difference in definition for the different lung regions, or a considerable deviation from the deposition distribution (approximately one third of the total lung dose in each region) does not really change this conclusion. This may have the consequence that the peripheral lung is underdosed with for instance antibiotics, which need to reach their minimum inhibitory concentration (MIC) value to become effective. In fact, not reaching the MIC value could result in bacterial resistance development and this is an aspect that will need serious consideration when changing from systemic to pulmonary administration for therapies against pulmonary infections. In this respect, also changing from approved formulation-device combinations to off-label combinations (e.g. in nebulisation) is potentially a risk when the delivered fine particle dose and the inhalation manoeuvre are not exactly the same.
6.4.8 Practical Implications for the Inhalation Manoeuvre
The optimal inspiratory manoeuvre for inhalation of a therapeutic aerosol depends on how it influences the aerosol generation process and the deposition pattern in the respiratory tract (Fig. 6.1). Its effect on the aerosol properties will be discussed in the next paragraphs in which the working principles of different aerosol generation devices are explained. From the deposition point of view particularly the (peak) flow rate, the inhaled volume and a certain breathhold pause are relevant, but good inhalation starts with exhalation to residual volume. As explained above, only with an inhalation from residual volume the alveoli can be reached effectively. Considering the dependence of inertial impaction in the oropharynx and first airway generations on the particle velocity, it may also be clear that a high flow rate during inhalation should be avoided, but the optimum in this respect depends on the performance of the aerosol generation device too. For good (dry powder) inhaler performance, even the acceleration to peak flow (flow increase rate) may be important. Inhalation should be continued until total lung capacity is reached. Premature stopping of the inhalation manoeuvre again has the consequence that the most distal airways are not reached with the aerosol, but it can also mean the total dose is not delivered. Once the smallest aerosol particles have reached the peripheral lung, they must stay there for a certain period of time to give sedimentation a chance and a breathhold pause of several seconds (preferably 5–10) is desired before starting exhalation.
6.5 Aerosol Generation Devices
Basically four different types of commercially available aerosol generation devices exist: dry powder inhalers (DPIs), metered-dose inhalers (MDIs), classic jet and ultrasonic nebulisers and a new class of high-performance liquid inhalers. Each of these categories has many different variations of the same basic design and working principle and they may also have significantly different performances. In the following paragraphs, firstly the conceptual design of the different types will be described and then their working principle will be explained. This has to be known to make the best possible choice for individual patients and to give an appropriate instruction for use. Only examples of specific devices will be discussed in more detail, because the still growing number of devices in each category is too extensive to make a complete survey.
6.5.1 Dry Powder Inhalers (DPIs)
DPIs are relatively new and their designs and working principles may not only be quite complex but also rather diverse between the different types, which easily leads to incorrect or suboptimal use. DPIs contain the active substance in the dry state which is beneficial from the viewpoint of stability. They can deliver much higher doses than MDIs and be disposable which is particularly desired for hygroscopic formulations of active substances, antibiotics against which bacterial resistance development has been reported and single-dose administrations such as vaccinations. Aerosol generation in DPIs is mostly breath activated, which eliminates the need for a good hand-lung coordination but requires the generation of sufficient flow rates and inhaled volumes to release a sufficiently high fine particle dose. DPIs have much higher airflow resistances than MDIs, which limits the attainable flow rate and by that, oropharyngeal deposition. Furthermore, DPIs can deliver higher fine particle fractions and finer aerosols at higher flow rates, which compensates to a certain extent for the increasing losses in the mouth, throat and upper airways when the patient inhales more forcefully, which results in an increased dominance of inertial particle impaction. DPIs exist not only in a large variety of different designs, they also have different performance properties and resistances to airflow. These differences in design and performance may be functional and have been chosen carefully to obtain the most optimal deposition of the active substance at the site of action. However, in many cases they may also be different for the same type (or class) of active substance(s), all having the same target area. These differences may lead to considerable variation in the delivered fine particle dose at this site of action. Differences in ease of handling, the flow manoeuvre needed and airflow resistance may be aspects to consider particularly for special patient groups, such as children and severe COPD patients. In the next paragraphs, the general design with some specific examples of DPIs will be presented and discussed in relation to their performances and the required operational procedures to obtain the best delivery of active substance.
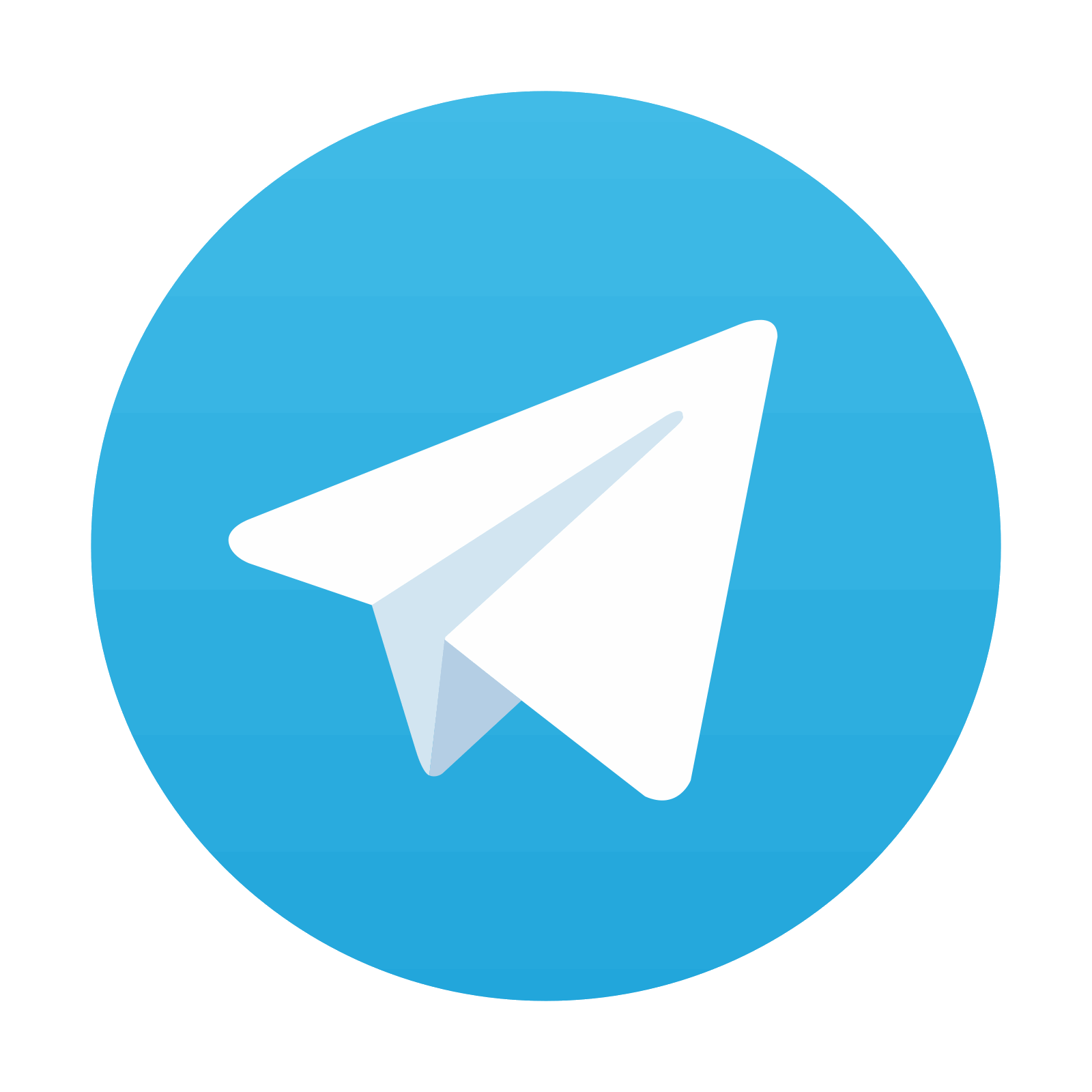
Stay updated, free articles. Join our Telegram channel
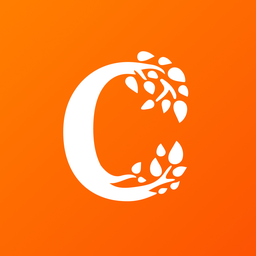
Full access? Get Clinical Tree
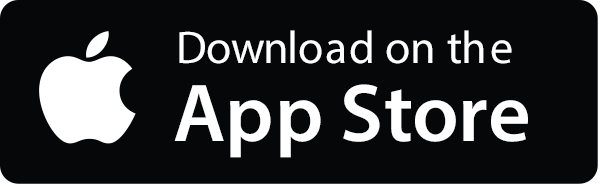
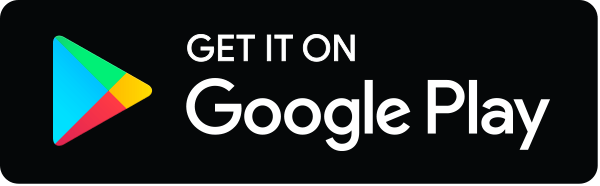