7.1 Proteins and Peptides for Inhalation
Proteins are polymers consisting of amino acids covalently linked by peptide bonds. Peptides are small proteins composed of up to a few dozen amino acids [1]. The molecular weights of larger proteins may range from thousands to several millions of atomic masses, depending on the number of amino acids in the chain [2]. Due to their large sizes, absorption through the epithelial barriers in the gastrointestinal tract is slow [3]. Furthermore, proteins are rapidly degraded by digestive enzymes. Thus oral bioavailability is generally poor. The most common route of administration for pharmaceutical proteins is parenteral, including intravenous, subcutaneous, and intramuscular injections. However, the pulmonary route has also been used for protein delivery because the lungs possess a large surface area and an extensive vascular network for absorption [4]. From a practical viewpoint, inhalation delivery is noninvasive and more convenient than injections. This is especially of value for pharmaceutical proteins that require long-term administration, such as insulin for the systemic treatment of diabetes mellitus or recombinant human deoxyribonuclease (rhDNase), also known as dornase alfa, for the local treatment of cystic fibrosis.
Many pharmaceutical proteins and peptides have been formulated as inhalation aerosols for local and systemic diseases (Table 7.1). The formulations are at various stages of development, ranging from Phase I studies to approved products. Exubera, for example, was a marketed insulin dry powder inhaler (DPI), but was withdrawn in October 2007 due to poor sales [5].
Table 7.1 Examples of proteins and peptides that have been applied as aerosols. Adapted from Cryan S-A. Carrier-based strategies for targeting protein and peptide drugs to the lungs. The AAPS Journal 2005;7(1):E20–E41. 7. Garcia-Contreras L, Smyth HDC. Liquid-spray or dry-powder systems for inhaled delivery of peptide and proteins? American Journal of Drug Delivery 2005;3(1):29–45.
Local diseases | Compounds |
α-1-antitrypsin deficiency | α-1-proteinase inhibitor |
Asthma | Anti-IgE Mab Interleukin-1R Interleukin-4 Lactoferrin |
Antituberculosis vaccine | Muramyl dipeptide |
Cancer/pneumocystis carnii | Interferon-γ Interleukin-2 |
Chronic bronchitis | Uridine triphosphate derivatives |
Cystic fibrosis | rhDNase (approved) Targeted genetics adeno-associated virus for cystic fibrosis |
Emphysema/cystic fibrosis | Alpha-1-antitrypsin Secretory leukoprotease inhibitor |
Lung transplant | Cyclosporine A |
Oxidative stress | Catalase Superoxide dismutase |
Respiratory distress syndrome | Surfactant proteins (approved) |
Systemic diseases | Compounds |
Anemia | Erythropoietin |
Anticoagulation | Heparin |
Cancer | Interleukins Luteinizing hormone-releasing hormone |
Diabetes insipidus | 1-deaminocysteine-8-D-arginine vasopressin |
Diabetes mellitus | Insulin (approved, but was later discontinued) |
Endometriosis | Leuprolide |
Growth hormone deficiency | Human growth hormone |
Hemophilia | Factor IX |
Multiple sclerosis | Interferon-β |
Neutropenia | Recombinant human granulocyte-colony stimulating factor (rhG-CSF) |
Osteoporosis | Calcitonin Parathyroid hormone |
Viral infections | Interferon-α Ribavirin |
7.1.1 Stability of Proteins
The biological activity of proteins is strongly dependent on their molecular structure, which involves several organizational levels [1]. The primary structure is the amino acid sequence, which ultimately dictates the noncovalent interactions the molecule undergoes in forming higher-order structures. The secondary structure is the periodic spatial arrangement of the polypeptide chain backbone due to hydrogen bonding between the C=O and N–H groups. Alpha-helices and beta-sheets are typical secondary structures within a protein. The tertiary structure is the three-dimensional conformation of the whole molecule, including the positions of all amino acid side chains. Some proteins may consist of multiple peptide chains grouped together by noncovalent intermolecular interactions. The arrangement of the subunits relative to each other constitutes the quaternary structure. Alterations in the protein structure at any level may lead to a change or loss in biological activity.
Protein degradation can be chemical or physical in nature. Chemical degradation involves changes in the covalent bonds in the polypeptide chain, mainly through hydrolysis, isomerization, deamidation, oxidation, and disulfide bridging [8]. Hydrolysis is the cleavage of peptide bonds, usually under extreme acidic or alkaline conditions. However, even under neutrality the asparagine–proline and asparagine–glycine bonds are relatively labile [3]. Except for glycine, all naturally occurring amino acids are chiral and belong to the L-form. They can isomerize to the D-form under certain circumstances [8]. Deamidation is the transformation of the amide groups in asparagine and glutamine side chains into carboxylic acids, forming aspartic and glutamic acids, respectively [8]. The side chains of cysteine, methionine, tyrosine, tryptophan, and histidine are prone to oxidation [8]. The sulfur-containing side chains of cysteine and methionine can also form intra- or intermolecular covalent disulfide bridges [8]. All chemical degradations will disrupt the primary protein structure.
Physical degradation refers to changes in the noncovalent interactions within or between protein molecules. It can occur independently from chemical degradation and results in alterations in the higher-order structures (secondary and above). Common types of physical degradation include denaturation, aggregation, precipitation, and adsorption [8]. Denaturation is the unfolding of a molecule from its native conformation. It may or may not be reversible. Denatured proteins may also associate with each other to form molecular aggregates [8]. Precipitation is essentially aggregation on a macroscopic scale, where dissolved proteins come out from solution due to a reduction in solubility [8]. Protein molecules are surface-active and may adsorb on to various interfaces [8].
Owing to their complex structure, proteins are much more fragile than small drug molecules. The risks of chemical and physical degradation depend on many physicochemical factors, such as temperature, pH, storage humidity, formulation constituents, delivery device, and manufacturing process, amongst others. These must be well studied and controlled to maintain the stability and efficacy of protein products.
7.1.2 Nebulizers and the AERx Pulmonary Delivery System
Protein nebule formulations are mainly aqueous solutions and are relatively easy to develop. The factors that may affect their stability are similar to those for general protein liquid formulations, such as ionic strength, pH, and the type and concentration of other dissolved compounds [9]. However, there are some potential problems that are unique to nebules. Atomizing air pressure, shear, recirculation, and progressive reduction of solution volume in jet nebulizers may lead to protein degradation [10]. Formulations without suitable protein stabilizers may also cause molecular adsorption to plastic surfaces [10]. The degradation rate of nebulized lactate dehydrogenase was found to increase with air pressure, nebulization time, and a low starting solution volume [11]. The aggregation and degradation rates of rhG-CSF also increased with nebulization time. However, these adverse effects were significantly reduced by the addition of polyethylene glycol (PEG) 1000 in the formulation [11]. This protective mechanism was attributed to the weak surface activity of PEG. The polymer may compete with the protein molecules to occupy the new liquid surfaces generated during nebulization, and consequently protect the proteins from degradation [11]. In another study, the stability of nebulized aviscumine, a dimeric protein, was improved by adding various surfactants, cryoprotectants, and buffer salts to the reconstitution medium [12–14].
High-energy vibrations and heat generated in ultrasonic nebulizers may denature proteins, as was observed on rhDNase [15, 16]. Therefore, only jet nebulizers are approved for the administration of the marketed dornase alfa product Pulmozyme [17]. The formulation of Pulmozyme is very simple, with only sodium chloride, calcium chloride dihydrate, and water for injection as excipients [17, 18]. This illustrates that not all protein nebule formulations require complex additives for stabilization. The stability of the protein of interest should be assessed on a case-by-case basis and excipients should be employed where appropriate.
The AERx pulmonary delivery system devised by Aradigm (California, USA) generates aerosols by mechanically extruding the liquid from a unit-dose reservoir through micron-sized orifices [19]. This device has been used for the delivery of a number of proteins, including rhDNase [20], insulin [21, 22], and interleukin-4 receptor [23].
7.1.3 Metered-Dose Inhalers (MDIs)
The major issue in metered-dose inhaler (MDI) formulations of proteins is the conformational stability of the macromolecules in liquefied propellants, which are less polar than water. Fourier-transform Raman spectroscopy has been applied to investigate the secondary structure of hen egg lysozyme suspended in HFA 134a and HFA 227 [24]. Conformational data on the peptide backbone, C-C stretching, and disulfide bonds were obtained. The technique is simple and nondestructive, as the formulations can be analyzed in crimped glass vials [24].
Various proteins have been formulated as MDI suspensions [25, 26]. Surfactants that are soluble in propellant were used to improve the suspendibility of the particles [25, 26]. These included Triton X-100, Triton X-405, Laureth 9, Brij 30, Brij 97, Brij 98, Tween 80, Nonidet-40, and diethylene glycol monoethyl ether for HFA 134a [25, 26]. An aqueous solution of the protein and surfactant was first freeze-dried to produce the required particles, which were subsequently suspended in HFA 134a alone or in a mixture of this propellant and dimethylether [25] or ethanol [26]. The propellant mixtures were found to improve the aerosol performance over the pure propellant. Kos Pharmaceuticals (Florida, USA) has developed an insulin MDI suspension in HFA 134a [27, 28]. This formulation has been shown to provide comparable blood-glucose control to subcutaneous insulin injections in Type 2 diabetic patients [27].
Compared to MDI suspensions, it is more difficult to develop solutions for proteins due to the nonpolar nature of the propellants. Nevertheless, a stable HFA 134a solution containing leuprolide acetate was successfully formulated incorporating water and an undisclosed cosolvent [29].
7.1.4 Dry Powder Inhalers (DPIs)
Formulating protein powders for pulmonary delivery is challenging as it requires not only flowability and dispersibility of the powders but also stability of the proteins. To satisfy the latter requirement, proteins are usually formulated as amorphous glasses, which are, however, physically unstable and tend to crystallize with interparticulate bond formation and loss of powder dispersibility. In addition, the stability requirements limit the manufacturing processes that can be used for protein powder production.
During powder production, removal of water from the proteins can cause significant molecular conformational damage, which can lead to further chemical degradation including aggregation, deamidation, and oxidation during storage. Amorphous glassy excipients, mainly carbohydrates, have been widely used to stabilize proteins for inhalation, such as lactose for rhDNase [30, 31]; trehalose, lactose, and mannitol for recombinant humanized anti-IgE monoclonal antibody (rhuMAbE25) [32]; and mannitol and raffinose for insulin [33]. Other suitable excipients include polymers (e.g. polyvinylpyrrolidone), proteins (e.g. human serum albumin), peptides (e.g. aspartame), amino acids (e.g. glycine), and organic salts (e.g. citrates). Although lactose has been widely used for inhalation products for small-molecule drugs, it may not be compatible with proteins. Being a reducing sugar, lactose is reactive toward lysine, and protein glycation has indeed been found in both rhDNase and rhuMAbE25 [32, 34]. The exact mechanism for protein stabilization by excipients is as yet unclear. However, contributing factors may include (1) formation of a glassy state of the protein–excipient system, (2) hydrogen bonding between the protein and excipient molecules, (3) crystallinity of the excipients, and (4) residual water content. The diffusion rate and mobility of the protein molecules in the glassy state are much lower than those in the rubbery state. Therefore, any physicochemical reactions leading to chemical instability will be reduced [35]. Crystalline excipients such as mannitol are known to decrease the stability of proteins [36]. However, mannitol can be used in the amorphous form, for instance, in the presence of glycine [37]. Fourier-transform infrared spectroscopy offers information on protein secondary structures and has provided evidence for protein stabilization by hydrogen bonding [38]. Water promotes instability of proteins by enhancing molecular mobility [39], as shown by nuclear magnetic resonance (NMR) spectroscopy [40]. The crystalline or amorphous state of the excipients is crucial because it controls the distribution of water between the protein and the excipient in a powder [41].
While glassy materials are desirable for protein chemical stability, an immediate drawback is physical instability. Moisture uptake by fine particles of hydrophilic amorphous materials can be very fast, due to their large specific surface area and high energy state. For example, water uptake by spray-dried rhDNase with lactose induced crystallization that adversely affected powder dispersibility [30, 31]. During crystallization, water acts as a plasticizer to lower the glass-transition temperature (Tg) (about 10 °C reduction per 1% water in sugar-containing formulations), which will enhance the molecular mobility required for nucleation if the Tg approaches the storage temperature [42]. It is thus important to keep the powder dry in order to maintain the high Tg, or to use excipients with a high Tg, or to store the powders at a low temperature. It has been proposed to store fragile glasses 50 °C below the Tg to minimize crystallization [43]. This method would be practical for room-temperature storage for amorphous materials with a Tg above 70 °C. It should be noted that the effect of moisture on powder dispersion can be instantaneous [44]. The hygroscopic effect can be reduced by using hydrophobic excipients, such as L-isoleucine [45].
Amorphous materials are usually cohesive, because they have higher surface energy and are more hygroscopic than the crystalline forms. Thus powder dispersibility is strongly dependent on the solid state. Most protein drugs are formulated with excipients for improved chemical stability, as discussed above. However, the distribution of protein and excipient(s) in a particle may not be uniform. When a protein–excipient solution droplet undergoes drying to form a particle, the outer surface tends to be enriched with proteins or macromolecules that are surface-active, while the small-molecule excipients diffuse rapidly into the particle core. Under special circumstances, small excipient molecules can crystallize on the particle surface [46]. Various formulation approaches have been employed to improve protein powder dispersibility. The particle size distribution can be controlled to decrease cohesion [46]. Proteins can be co-spray-dried with a suitable excipient to modify the surface energy and morphology; for example, rhDNase with sodium chloride [46]. Blending of protein drugs with inert carrier particles (e.g. lactose) can improve dispersibility [46]. Large (mean diameter >5 μm) and small (3−5 μm; PulmoSphere) porous particles containing proteins have excellent aerosol performance due to their low cohesion and small aerodynamic diameters [47–49]. Surface corrugation can also decrease cohesion by reducing the interparticulate contact areas. Wrinkled, nonporous, solid bovine serum albumin particles were reported to disperse significantly better than their nonwrinkled, spherical counterparts [50–52].
Inhalable protein powders can be produced by various methods, including spray-drying [49, 53–55], spray freeze-drying [48], lyophilization followed by milling [56], and solvent precipitation with supercritical fluids [57–60]. Precipitation with regular antisolvents can be carried out using high-gravity controlled precipitation [61–63] or confined impinging jet-mixing [64, 65]. Details on these processes are expounded in Chapter 4. Some issues regarding these techniques that are particularly relevant to protein formulations need to be noted. Spray-drying exposes the protein to mechanical shear and air–liquid interfacial denaturation. The hot air for drying also subjects the protein to thermal stress and denaturation. Aggregation of spray-dried recombinant human growth hormone was suppressed by adding Zn2+ ions and surfactant polysorbate 20 [53, 54]. Compared to spray-drying, spray freeze-drying produces porous and light particles with superior dispersibility, and the production yield is almost 100%. However, this process is more time-consuming and expensive. Gas-jet-milling of lyophilized proteins can cause contamination and inactivation. Thus abrasive-resistant mills using high-purity nitrogen and milling stabilizers such as human serum albumin and sorbitol are required [56]. Supercritical carbon dioxide is a good antisolvent for precipitation because it is nontoxic, economical, and has a low critical temperature of 31.1 °C for operation. However, due to its nonpolar nature, it is not readily miscible with water. Special nozzles have been employed to enhance mixing with aqueous protein solutions [59, 60]. Alternatively, supercritical carbon dioxide modified with ethanol can be used as an antisolvent [57, 58]. Since carbon dioxide is acidic, the pH of the mixture should be controlled to avoid protein degradation. Excipients such as PEG and self-assembling small organic molecules can be combined with proteins to form microparticles by precipitation [66–68].
7.2 Controlled-Release Formulations for Inhalation
Two significant drawbacks of current inhalation therapies are the short duration of action and the need to deliver drugs at least three to four times daily [69]. Despite the increasing number of inhalation formulations for the treatment of respiratory illnesses, no controlled-release inhalation system exists to date. Maximizing the concentration/dose ratio in the lung tissue by delivering the medicament to the upper airways will potentially decrease systemic exposure [70]. In addition, by promoting systemic absorption by delivering the inhalation dose to the lower respiratory tract, the delivery of systemic agents becomes feasible [71].
Many drug molecules have been investigated for their potential as local controlled-release agents in the lung, including antibacterials [72, 73], antivirals [74, 75], antifungals [76–78], cytotoxic agents [73], and immunosuppressives [79]. In addition, by prolonging the presence of β2-agonists (as asthma is influenced by circadian rhythm), the exacerbation of asthma during sleep may potentially be reduced [80]. Insulin, rhDNase, α-1-antitrypsin, DNA vaccines, interferons, calcitonin, human growth hormone, parathyroid hormone, vaccines, gene therapy, and leuprolide are just a few examples of systemic molecules that are candidates for systemic controlled-release inhalation therapies [81–84]. Another example is morphine, a small painkiller molecule, which would be extremely advantageous for cancer patients or for postoperative pain management if formulated in a controlled-release inhalation formulation [85].
7.2.1 Challenges Facing Controlled-Release Inhalation Therapy
The controlled-release formulations have complex challenges which add to the requirements of formulating any inhalation therapy. Not only should the particles be inhalable (aerodynamic diameter <5 μm), but a release-modifying excipient is needed for controlled release of the drug after delivery. Both of these steps are challenging: the production of micron-sized particles is difficult, since with a reduction in size there is a significant increase in surface area-to-mass ratio [86–88]; subsequently, with an increase in surface area, it becomes more difficult to produce a controlled-release profile and incorporate effective release agents.
The physiology of the lung and its impact on resident particles is another hurdle. The deposited particles will be primarily removed by the mucociliary escalator towards the pharynx and ultimately deposited in the gastrointestinal tract within 24 hours [89], if the aerodynamic particle size of a formulation is in the range 2.5–6.0 μm (as for local therapy). Moreover, some of these particles may be absorbed though the epithelium in this region into the blood or the lymphatic system [90].
Alternatively, insoluble particles deposited in the alveolar sac are subjected to clearance mainly via alveolar macrophages. These exist in numbers equivalent to five to seven macrophages per alveolus [91]. They engulf and enzymatically degrade foreign particulate matter or microorganisms, and migrate the results to the mucociliary escalator or lymph tissue [92] in a time frame of weeks to months [89]. This is the case when particles have an aerodynamic diameter <2.5 μm, as for systemic delivery, where the particles will be primarily deposited in the alveoli [93]. In Section 7.2.2, the different strategies used to produce controlled-release formulations for inhalation are highlighted.
7.2.2 Production of Inhalable Controlled-Release Formulations
Biodegradable Excipient-Based Matrices
These matrices range from synthetic to natural materials. Examples include biocompatible synthetic polymers such as polylactic co-glycolic acid (PLGA), polylactic acid (PLA), PEG, and polyvinyl alcohol (PVA), and natural polymers or proteins such as chitosan and albumin.
These systems depend on the poor solubility of the polymer or drug–polymer interaction to affect the drug release. When drug molecules are incorporated into these biodegradable matrices and deposited at the air–liquid interface in the lung, they slowly release the active drug. The drug then passively diffuses out of the particulate to be slowly released into the systemic circulation [75, 94, 95].
PLGA and PLA have been the most commonly reported polymers utilized for potential respiratory sustained-release systems. Although they are usually prepared via double emulsion formation followed by solvent evaporation [96, 97], spray-drying fluidized bed granulation was used by Yamamoto et al. for the production of PLGA nanocomposite granules [98]. In this study, insulin-loaded nanospheres with mannitol showed significantly decreased blood glucose levels as well as prolonged pharmacological effects due to the preferable inhalation performance and gradual release of insulin from nanospheres in the nanocomposite.
Production of porous, low-density, respirable particles were also achieved by spray-drying active ingredients dissolved in ethanolic solution with other excipients [99–101]. PLGA and PLA were used to form nonbrittle, shell-like, large particles utilizing this method for controlled-release powder production. Edwards et al. produced large-size (>5 μm), low-density (<0.4 g/cm3) particles of both insulin and testosterone with PLGA and PLA [47]. The porous particles were inhaled deep into the lungs and were detected for longer when compared with the conventional particles (96 hours compared to 4 hours, respectively).
Large porous particles were also prepared using a supercritical CO2 treatment process. This method was used to prepare large porous deslorelin-PLGA particles with reduced residual solvent content, retained deslorelin integrity, sustained release, and reduced cellular uptake [102].
Highly porous large PLGA microparticles were recently produced using ammonium bicarbonate as an effervescent porogenic agent. These resultant particles had suitable aerodynamic properties and good encapsulation efficiency, in addition to a reduced macrophage uptake and a sustained release pattern of doxorubicin [103].
PVA has also been studied as a potential biocompatible polymer for pulmonary delivery. PVA was found to improve the aerosolization efficiency of spray-dried disodium cromoglycate (DSCG) and to prolong the release of the active drug from the microparticles [104, 105]. Bovine serum albumin microparticles for pulmonary inhalation were also produced using PVA, and similarly showed prolonged release profiles [106]. Interestingly, another study by Liao et al. showed that the use of PVA in the formulation of pressurized MDIs physically stabilized suspensions of test proteins by limiting the potential for irreversible aggregation [107].
It is important to note that in spite of the popularity and the success of using polymeric release-modifying agents, the long residence time due to slow degradation might lead to pulmonary accumulation of these polymers, especially with daily administration [108, 109]. Furthermore, pulmonary administration of PLA microspheres to rabbits was associated with raised neutrophil count, inflammation at sites close to microparticle deposition, and hemorrhage [110]. In addition, in cell-based toxicity screening, PLGA and PLA significantly reduced cell viability when compared to lipid-based particles [111]. A recent comparative study of a series of potential polymers has shown that PLGA had the greatest toxicity of the polymers when studied on the Calu-3 monolayers [112], in comparison with a preliminary A549 cell toxicity study, which indicated that PVA had limited effect on cell viability after 24 hours’ exposure [106]. While the same study by Sivadas et al. showed that hydroxypropyl cellulose had high delivery efficiency, sodium hyaluronate and chitosan showed low toxicity and controlled-release behavior, and ovalbumin and chitosan had improved systemic delivery of a model protein-loaded particle system prepared by spray-drying.
Avoidance of mucociliary clearance and potentially prolonged residence time through physical adsorption may be achieved by the use of mucoadhesive polymers such as chitosan [113, 114] and hydroxypropyl cellulose [115]. Chitosan is also an absorption enhancer as it has been reported to loosen the tight junctions between the epithelial cells and thus facilitate absorption [116, 117]. Surface modification of PLGA nanospheres using chitosan as a mucoadhesive coat has been reported to lead to slower elimination from the lungs and to enhance the absorption of the active drug compared to unmodified PLGA nanospheres [117]. Co-spray-dried chitosan formulations containing either soluble terbutaline sulfate or insoluble beclomethasone dipropionate drug molecules had significantly longer release rates than the drug alone [118, 119]. Formulations containing chitosan and beclamethasone dipropionate showed in vitro respiratory efficiencies >43% and up to 12 hours’ release in 1000 mL phosphate buffer [118]. Furthermore, the release of encapsulated insulin could be controlled by incorporating a negatively charged phospholipid in a film coating chitosan nanoparticles [120].
To overcome potential toxicity and accumulation of these polymers in the lung, the use of natural protein carriers may be a suitable alternative. Albumin incorporation in the formulation of DPIs is expected to prolong the release of particles in the alveoli [121]. Albumin microspheres were reported to deliver tetrandine [122] and were found to provide greater drug concentrations in the lungs when compared to conventional oral dosing. Kwon et al. prepared bovine serum albumin porous microparticles using sucrose ethyl isobutylate as an additive for sustained protein release and a cyclodextrin derivative as a porogen; the in vitro release was observed up to 7 days [123]. In another study by Li et al., albumin microspheres entrapping an antibacterial drug showed in vitro sustained-release profiles for over 12 hours [121]. Addition of albumin may also have a dramatic effect on the morphology and fine-particle fraction of the spray-dried powders [124].
Examples of natural gums being studied as potential release-modifying agents are xanthan and locust bean gums. These gums were shown to reduce the release of salbutamol, indicating that a strong gel was formed by the intermolecular interaction between xanthan and locust bean gums [125].
Increasing the total molecular weight of the system and hence retarding the rate of absorption of the active ingredient across the epithelium of the alveoli can be achieved by forming conjugates using large-molecular-mass polymers or proteins [94]. PEG is a macromolecule that has been conjugated with proteins (e.g. asparaginase) [94, 126]. These conjugates can be conveniently fabricated to present a variety of ligands at the distal ends of spacer PEG chains [127, 128]. Using calcium phosphate–PEG particles, the bioavailability and duration of action of insulin were enhanced when administered to the lungs of rats compared to conventional subcutaneous delivery [129].
It is important to note that attaching polymeric materials to proteins requires careful understanding of the positions of the functional groups so as not to block the active protein site and provoke loss of activity.
Molecular Dispersions
A liposomal vesicle’s size—ranging from around 20 nm to several microns—surface charge, number of bilayers, and method of preparation are the major parameters controlling its half-life and the extent of drug entrapment [130]. Furthermore, since liposomes can be formulated from different types of lipid, it is possible to produce a wide range of physicochemical properties that can accommodate a wide range of encapsulated drug molecules. Liposomal sizes of 50–200 nm are considered to be optimal for avoiding phagocytosis by macrophages while still being useful for encapsulating drugs [131]. Liposomal and phospholipid-based liquid formulations have been used for the treatment of neonatal respiratory distress syndrome and seasonal asthma [132], thus making them relatively safe vehicles [133] for controlled-release applications, in comparison with the biodegradable polymeric matrices.
In addition, different surface charges and bilayer fluidities, depending on the production conditions and chemical composition, can be manipulated for a desired physiological effect. Cationic liposomes have been used to bind negatively charged DNA and fuse to cell membranes for the treatment of cystic fibrosis [134, 135]. Such an approach, in spite of decreasing transfection efficiencies, avoids the immunogenic response and risks associated with the use of viral vectors [136].
Liposomal formulations have generally been found to reduce systemic side effects and improve clinical effects. The effect of the liposomal encapsulation of bronchodilators (sympathomimetic amines), anti-asthma drugs (sodium cromoglycate), antimicrobial agents (amikacin, benzylpenicillin, oxytocin), antiviral agents (enviroxine), cytotoxic agents (β-cytosine arabinoside), and antioxidant agents (catalase, superoxide dismutase, glutathione) has been summarized by Zeng et al. [75]. When insulin is encapsulated in liposomes, its absorption from the lungs is enhanced and prolonged [75].
Significantly higher drug levels and prolonged drug retention in the respiratory tract have been observed in a liposomal formulation by jet nebulization when compared to free ciprofloxacin [137]. In this study, aerosol inhalation of liposome-encapsulated ciprofloxacin, given either prophylactically or therapeutically, provided complete protection to mice against a pulmonary lethal infection model of F. tularensis. In contrast, ciprofloxacin given in its free form was ineffective.
More recently, Stark et al. encapsulated a vasoactive intestinal peptide into unilamellar liposomes [138]. They found that sterically stabilized liposomal formulations have the potential to enhance the life-span and biological activity of peptide drugs in the metabolic environment of the lung. Chono et al. indicated that, according to pharmacokinetic/pharmacodynamic analysis, the pulmonary administration of mannosylated ciprofloxacin-liposomes exhibited potent antibacterial effects against many bacteria tested and could be useful against intracellular parasitic infections [139].
A modification of the liposomal system is agglomerated vesicle technology. In vivo cleavage of the agglomerated liposomes, which are used as core nanoparticles, results in controlled release of the drug [127, 128, 140].
Solid Lipid Particles
Formulating liposomes into dry powders [141, 142] may be a good alternative for overcoming the high production cost, disruption, and loss of entrapped medicaments during storage or nebulization which remain significant challenges in the production and stability of liposomes [143, 144]. These systems are more chemically and physically stable than liposomes, have good entrapment yields, use inexpensive carriers, and avoid toxic solvent residues [145]. Among the limited number of reports available in this field, Jaspart et al. pointed to salbutamol acetonide-loaded solid lipid nanoparticles of glyceryl behenate [146]; these formulations showed delayed release profiles in vitro when compared to the free drug or the physical mixtures, with no significant inflammatory airway response observed in similar systems after intratracheal administration in rats [147].
Coating or encapsulating drug particles in a lipid outer shield was found to decrease microparticle uptake by macrophages. The uptake of PLGA microparticles by cultured macrophages was found to be significantly reduced when dipalmitoylphosphatidylcholine was incorporated into the formulation [148]. Furthermore, the in vitro release of the incorporated drugs was also retarded in this case. Cook et al. demonstrated reduced in vitro release rates of terbutaline sulfate when the primary particles were coated with a hydrogenated palm oil and dipalmitoylphosphatidylcholine lipid system [141]. Similarly, chitosan nanoparticles coated with a lipid film of dipalmitoylphosphatidylcholine and dimyristoylphosphatidylcholine were successful in controlling the release of insulin from the nanoparticles under in vitro conditions [120].
Viscous Systems
Forming a gel interface for the passive transport of the uniformly dispersed drug through the gel matrix upon deposition in the lung is the principle of this approach. Pulmonary absorption of 5(6)-carboxyfluorescein was regulated using 5% gelatine, 1% PVA, 1% hydroxypropyl cellulose, 1% methyl cellulose 400, or 1% hyaluronic acid [149]. Intratracheal administration of these aqueous solutions had a significant effect on drug plasma levels in rats. On the other hand, the excipients’ effect on the release profile appeared to be drug-specific, since the release rate of fluorescein isothiocyanate-labeled dextrans was not regulated by gelatine or PVA. Similarly, iota- and kappa-carrageenans have been shown to be potential release-modifying polysaccharide gels. A modified absorption rate of theophylline and flutecasone propionate was observed when the polymers were utilized in solutions <5% w/v, with no evident damaging or inflammatory effect on lung tissue [150].
It is interesting to note that all these approaches have shown successful release profiles either in vitro (i.e. using different conventional and modified dissolution methodologies) or in vivo (i.e. in murine models). However, the methodologies and approaches for evaluating these systems are diverse. Salama et al. have compared different in vitro methods in use to evaluate the release profiles of controlled-release DPIs and found significant differences with different approaches [105]. Without a standardized method of assessing and testing controlled-release formulations for inhalation, it becomes quite difficult to accurately compare methods of production and their effectiveness. Although many challenges exist, controlled-release formulations to the lung have not yet reached their full potential and are still underappreciated.
1. Campbell MK. Biochemistry. Orlando, FL: Harcourt Brace & Company; 1999.
2. Bailey PSJr, Bailey CA. Organic Chemistry: A Brief Survey of Concepts and Applications. Upper Saddle River, NJ: Prentice-Hall International; 1995.
3. Crommelin D, van Winden E, Mekking A. Delivery of pharmaceutical proteins. In: Aulton ME, editor. Pharmaceutics: The Science of Dosage Form Design. Edinburgh: Churchill Livingstone; 2002. pp. 544–553.
4. Qiu Y, Gupta PK, Adjei AL. Absorption and bioavailability of inhaled peptides and proteins. In: Adjei AL, Gupta PK, editors. Inhalation Delivery of Therapeutic Peptides and Proteins. New York, NY: Marcel Dekker; 1997. pp. 89–131.
5. Bailey CJ, Barnett AH. Why is Exubera being withdrawn? British Medical Journal 2007;335:1156.
6. Cryan S-A. Carrier-based strategies for targeting protein and peptide drugs to the lungs. AAPS Journal 2005;7(1):E20–E41.
7. Garcia-Contreras L, Smyth HDC. Liquid-spray or dry-powder systems for inhaled delivery of peptide and proteins? American Journal of Drug Delivery 2005;3(1):29–45.
8. Manning MC, Patel K, Borchardt RT. Stability of protein pharmaceuticals. Pharmaceutical Research 1989;6(11):903–918.
9. Arakawa T, Prestrelski SJ, Kenney WC, Carpenter JF. Factors affecting short-term and long-term stabilities of proteins. Advanced Drug Delivery Reviews 1993;10:1–28.
10. Gupta PK, Adjei AL. Therapeutic inhalation aerosols. In: Adjei AL, Gupta PK, editors. Inhalation Delivery of Therapeutic Peptides and Proteins. New York, NY: Marcel Dekker; 1997. pp. 185–234.
11. Niven RW, Ip AY, Mittelman SD, Farrar C, Arakawa T, Prestrelski SJ. Protein nebulization: I. Stability of lactate dehydrogenase and recombinant granulocyte-colony stimulating factor to air-jet nebulizaion. International Journal of Pharmaceutics 1994;109:17–26.
12. Steckel H, Eskander F, Witthohn K. Effect of excipients on the stability and aerosol performance of nebulised aviscumine. Journal of Aerosol Medicine 2003;16:417–432.
13. Steckel H, Eskander F, Witthohn K. Effect of cryoprotectants on the stability and aerosol performance of nebulized aviscumine, a 57-kDa protein. European Journal of Pharmaceutics and Biopharmaceutics 2003;56:11–21.
14. Steckel H, Eskander F, Witthohn K. The effect of formulation variables on the stability of nebulised aviscumine. International Journal of Pharmaceutics 2003;257:181–194.
15. Phipps PR, Gonda I. Droplets produced by medical nebulizers: some factors affecting their size and solute concentration. Chest 1990;97:1327–1332.
16. Cipolla DC, Clark AR, Chan H-K, Gonda I, Shire SJ. Assessment of aerosol delivery systems for recombinant human deoxyribonuclease. STP Pharma Sciences 1994;4:50–62.
17. Roche, Products. Pulmozyme® Consumer Medicine Information. Dee Why, NSW, Australia: Roche Products Pty Ltd; 2008.
18. Gonda I. Deoxyribonuclease inhalation. In: Adjei AL, Gupta PK, editors. Inhalation Delivery of Therapeutic Peptides and Proteins. New York, NY: Marcel Dekker; 1997. pp. 355–365.
19. Rubsamen R. Novel aerosol peptide drug delivery systems. In: Adjei AL, Gupta PK, editors. Inhalation Delivery of Therapeutic Peptides and Proteins. New York, NY: Marcel Dekker; 1997. pp. 703–731.
20. Mudumba S, Khossravi M, Yim D, Rossi T, Pearce D, Hughes M, et al. Delivery of rhDNase by the AERx® pulmonary delivery system. In: Dalby RN, Byron PR, Farr S, Peart J, editors. Respiratory Drug Delivery VII. Raleigh, NC: Serentec Press; 2000. pp. 329–332.
21. Henry RR, Mudaliar SRD, Howland WC III, Chu N, Kim D, An B, et al. Inhaled insulin using the AERx Insulin Diabetes Management System in healthy and asthmatic subjects. Diabetes Care 2003;26:764–769.
22. Farr S, Reynolds D, Nat A, Srinivasan S, Roach M, Jensen S, et al. Technical development of AERx® Diabetes Management System: essential characteristics for diabetes treatment with pulmonary insulin. In: Dalby RN, Byron PR, Peart J, Farr S, editors. Respiratory Drug Delivery VIII. Raleigh, NC: Davis Horwood International; 2002. pp. 51–60.
23. Sangwan S, Agosti JM, Bauer LA, Otulana BA, Morishige RJ, Cipolla DC, et al. Aerosolized protein delivery in asthma: gamma camera analysis of regional deposition and perfusion. Journal of Aerosol Medicine 2001;14:185–195.
24. Quinn ÉÁ, Forbes RT, Williams AC, Oliver MJ, McKenzie L, Purewal TS. Protein conformational stability in the hydrofluoroalkane propellants tetrafluoroethane and heptafluoropropane analysed by Fourier transform Raman spectroscopy. International Journal of Pharmaceutics 1999;186:31–41.
25. Brown AR, George DW. Tetrafluoroethane (HFC 134A) propellant-driven aerosols of proteins. Pharmaceutical Research 1997;14:1542–1547.
26. Williams RO III, Liu J. Formulation of a protein with propellant HFA 134a for aerosol delivery. European Journal of Pharmaceutical Sciences 1998;7:137–144.
27. Hausmann M, Dellweg S, Osborn C, Heinemann L, Buchwald A, Rosskamp R, et al. Inhaled insulin as adjunctive therapy in subjects with type 2 diabetes failing oral agents: a controlled proof-of-concept study. Diabetes, Obesity and Metabolism 2006;8:574–580.
28. Adjei AL, Genova P, Zhu Y, Sexton F. Method of treating a systemic disease. US patent 7056494. 2006.
29. Brambilla G, Berrill S, Davies RJ, Ganderton D, George SC, Lewis DA, et al. Formulation of leuprolide as an HFA solution pMDI. Journal of Aerosol Medicine 2003;16:209.
30. Chan H-K, Gonda I. Solid state characterization of spray-dried powders of recombinant human deoxyribonuclease (rhDNase). Journal of Pharmaceutical Sciences 1998;87(5):647–654.
31. Clark AR, Dasovich N, Gonda I, Chan H-K. The balance between biochemical and physical stability for inhalation protein powders: rhDNase as an example. In: Dalby RN, Byron PR, Farr S, editors. Resiratory Drug Delivery V. Buffalo Grove, IL: Interpharm Press; 1996. pp. 167–174.
32. Andya JD, Maa Y-F, Constantino HR, Nguyen P-A, Dasovich N, Sweeney TD, et al. The effect of formulation excipients on protein stability and aerosol performance of spray-dried powders of a recombinant humanized anti-IgE monoclonal antibody. Pharmaceutical Research 1999;16:350–358.
33. Patton JS, Foster L, Platz RM. Methods and compositions for pulmonary delivery of insulin. US patent 5997848. 1999.
34. Quan CP, Wu S, Dasovich N, Hsu C, Patapoff T, Canova-Davis E. Susceptibility of rhDNase I to glycation in the dry-powder state. Analytical Chemistry 1999;71:4445–4454.
35. Frank F. Long-term stabilization of biologicals. Biotechnology 1994;12:253–256.
36. Izutsu K-I, Yoshioka S, Terao T. Decreased protein-stabilizing effects of cryoprotectants due to crystallization. Pharmaceutical Research 1993;10:1232–1237.
37. Pikal MJ, Dellerman KM, Roy ML, Riggin RM. The effects of formulation variables on the stability of freeze-dried human growth hormone. Pharmaceutical Research 1991;8:427–436.
38. Carpenter JF, Prestrelski SJ, Dong A. Application of infrared spectroscopy to development of stable lyophilized protein formulations. European Journal of Pharmaceutics and Biopharmaceutics 1998;45:231–238.
39. Hageman MJ. Sorption and solid-state stability of proteins. In: Ahern TJ, Manning MC, editors. Stability of Protein Pharmaceuticals, Part A: Chemical and Physical Pathways of Protein Degradation. New York, NY: Plenum Press; 1992. pp. 273–309.
40. Separovic F, Lam YH, Ke X, Chan H-K. A solid-state NMR study of protein hydration and stability. Pharmaceutical Research 1998;15:1816–1821.
41. Chan H-K, Au-Yeung K-L, Gonda I. Development of a mathematical model for the water distribution in freeze-dried solids. Pharmaceutical Research 1999;16:660–665.
42. Roos Y, Karel M. Plasticizing effect of water on thermal behavior and crystallization of amorphous food models. Journal of Food Science 1991;56:38–43.
43. Hancock BC, Shamblin SL, Zografi G. Molecular mobility of amorphous pharmaceutical solids below their glass transition temperatures. Pharmaceutical Research 1995;12:799–806.
44. Chew NYK, Chan H-K. Effect of humidity on the dispersion of dry powers. In: Dalby RN, Byron PR, Farr S, Peart J, editors. Respiratory Drug Delivery VII. Raleigh, NC: Serentec Press; 2000. pp. 615–618.
45. Yamashita C, Nishibayashi T, Akashi S, Toguchi H, Odomi M. A novel formulation of dry powder for inhalation of peptides and proteins. In: Dalby RN, Byron PR, Farr S, editors. Respiratory Drug Delivery V. Buffalo Grove, IL: Interpharm Press; 1996. pp. 483–486.
46. Chan H-K, Clark A, Gonda I, Mumenthaler M, Hsu C. Spray dried powders and powder blends of recombinant human deoxyribonuclease (rhDNase) for aerosol delivery. Pharmaceutical Research 1997;14:431–437.
47. Edwards DA, Hanes J, Caponetti G, Hrkach J, Ben-Jebria A, Eskew ML, et al. Large porous particles for pulmonary drug delivery. Science 1997;276(5320):1868–1872.
48. Maa Y-F, Nguyen P-A, Sweeney TD, Shire SJ, Hsu CC. Protein inhalation powders: spray drying vs spray freeze drying. Pharmaceutical Research 1999;16:249–254.
49. Bot AI, Tarara TE, Smith DJ, Bot SR, Woods CM, Weers JG. Novel lipid-based hollow-porous microparticles as a platform for immunoglobulin delivery to the respiratory tract. Pharmaceutical Research 2000;17:275–283.
50. Chew NYK, Chan HK. Use of solid corrugated particles to enhance powder aerosol performance. Pharmaceutical Research 2001;18(11):1570–1577.
51. Chew NYK, Tang P, Chan HK, Raper JA. How much particle surface corrugation is sufficient to improve aerosol performance of powders? Pharmaceutical Research 2005;22(1):148–152.
52. Adi S, Adi H, Tang P, Traini D, Chan H-K, Young PM. Micro-particle corrugation, adhesion and inhalation aerosol efficiency. European Journal of Pharmaceutical Sciences 2008;35:12–18.
53. Mumenthaler M, Hsu CC, Pearlman R. Feasibility study on spray-drying protein pharmaceuticals: recombinant human growth hormone and tissue-type plasminogen activator. Pharmaceutical Research 1994;11:12–20.
54. Maa Y-F, Nguyen P-AT, Hsu SW. Spray-drying of air-liquid interface sensitive recombinant human growth hormone. Journal of Pharmaceutical Sciences 1998;87:152–159.
55. Vanbever R, Mintzes JD, Wang J, Nice J, Chen D, Batycky R, et al. Formulation and physical characterization of large porous particles for inhalation. Pharmaceutical Research 1999;16:1735–1742.
56. Platz RM, Ip A, Whitham CL., Process for preparing micronized polypeptide drugs. US patent 5354562. 1994.
57. Bustami RT, Chan H-K, Dehghani F, Foster NR. Generation of micro-particles of proteins for aerosol delivery using high pressure modified carbon dioxide. Pharmaceutical Research 2000;17(11):1360–1366.
58. Bustami RT, Chan H-K, Foster NR. Aerosol delivery of protein powders processed by supercritical fluid technology. In: Dalby RN, Byron PR, Farr S, Peart J, editors. Respiratory Drug Delivery VII. Raleigh, NC: Serentec Press; 2000. pp. 611–614.
59. Sievers RW, Sellers SP, Kusek KD, Glark GS, Korte BJ. Fine-particle formation using supercritical carbon dioxide-assisted aerosolization and bubble drying. In: Proceedings of the 218th ACS National Meeting. New Orleans, LA; American Chemical Society: 1999.
60. Sloan R, Hollowood HE, Hupreys GO, Ashraf W, York P. Supercritical fluid processing: preparation of stable protein particles. In: Proceedings of the Fifth Meeting of Supercritical Fluids. Nice, France; 1998.
61. Chiou H, Li L, Hu T, Chan HK, Chen JF, Yun J. Production of salbutamol sulfate for inhalation by high-gravity controlled antisolvent precipitation. International Journal of Pharmaceutics 2007;331(1):93–98.
62. Chen J-F, Zhou M-Y, Shao L, Wang Y-Y, Yun J, Chew NYK, et al. Feasibility of preparing nanodrugs by high-gravity reactive precipitation. International Journal of Pharmaceutics 2004;269:267–274.
63. Hu T, Chiou H, Chan H-K, Chen J-F, Yun J. Preparation of inhalable salbutamol sulfate using reactive high gravity controlled precipitation. Journal of Pharmaceutical Sciences 2008;97:944–949.
64. Chiou H, Chan H-K, Prud’homme RK, Raper JA. Evaluation on the use of the confined liquid impinging jets for the synthesis of nanodrug particles. Drug Development and Industrial Pharmacy 2008;34:59–64.
65. Chiou H, Chan H-K, Heng D, Prud’homme RK, Raper JA. A novel production method for inhalable cyclosporine A powders by confined liquid impinging jet precipitation. Journal of Aerosol Science 2008;39:500–509.
66. Steiner SS, Pfützner A, Wilson BR, Harzer O, Heinemann L, Rave K. Technosphere/ insulin: proof of concept study with a new insulin formulation for pulmonary delivery. Experimental and Clinical Endocrinology & Diabetes 2002;110: 17–21.
67. Lian H, Steiner SS, Sofia RD, Woodhead JH, Wolf HH, White HS, et al. A self-complementary, self-assembling microsphere system: application for intravenous delivery of the antiepileptic and neuroprotectant compound felbamate. Journal of Pharmaceutical Sciences 2000;89:867–875.
68. Brown LR, Rashba-Step J, Scott TL, Qin Y, Rulon PW, McGeehan J, et al. Pulmonary delivery of novel insulin microspheres. In: Dalby RN, Byron PR, Peart J, Farr S, editors. Respiratory Drug Delivery VIII. Raleigh, NC: Davis Horwood International; 2002. pp. 431–434.
69. Byron PR. Prediction of drug residence times in regions of the human respiratory tract following aerosol inhalation. Journal of Pharmaceutical Sciences 1986;75(5):433–438.
70. Bennett WD, Brown JS, Zeman KL, Hu S-C, Scheuch G, Sommerer K. Targeting delivery of aerosols to different lung regions. Journal of Aerosol Medicine 2002;15(2):179–188.
71. Jain KK. Drug delivery systems—an overview. In: Jain KK, editor. Drug Delivery Systems. Totowa, NJ: Humana Press; 2008. pp. 1–50.
72. Poyner EA, Alpar HO, Almeida AJ, Gamble MD, Brown MRW. A comparative study on the pulmonary delivery of tobramycin encapsulated into liposomes and PLA microspheres following intravenous and endotracheal delivery. Journal of Controlled Release 1995;35(1):41–48.
73. Schreier H, Gonzalez-Rothi RJ, Stecenko AA. Pulmonary delivery of liposomes. Journal of Controlled Release 1993;24(1–3):209–223.
74. Taylor KM, Newton JM. Liposomes for controlled delivery of drugs to the lung. Thorax 1992;47(4):257–259.
75. Zeng XM, Martin GP, Marriott C. The controlled delivery of drugs to the lung. International Journal of Pharmaceutics 1995;124(2):149–164.
76. Gilbert BE. Liposomal aerosols in the management of pulmonary infections. Journal of Aerosol Medicine 1996;9(1):111–122.
77. Ruijgrok EJ, Vulto AG, Van Etten EW. Aerosol delivery of amphotericin B desoxycholate (Fungizone) and liposomal amphotericin B (AmBisome): aerosol characteristics and in-vivo amphotericin B deposition in rats. Journal of Pharmacy and Pharmacology 2000;52(6):619–627.
78. Alvarez CA, Wiederhold NP, McConville JT, Peters JI, Najvar LK, Graybill JR, et al. Aerosolized nanostructured itraconazole as prophylaxis against invasive pulmonary aspergillosis. Journal of Infection 2007;55(1):68–74.
79. Behr J, Zimmermann G, Baumgartner R, Leuchte H, Neurohr C, Brand P, et al. Lung deposition of a liposomal cyclosporine a inhalation solution in patients after lung transplantation. Journal of Aerosol Medicine and Pulmonary Drug Delivery 2009;22(2):1–9.
80. Smolensky MH, D’Alonzo GE, Kunkel G, Barnes PJ. Day-night patterns in bronchial patency and dyspnea: basis for once-daily and unequally divided twice-daily theophylline dosing schedules. Chronobiology International 1987;4(3):303–307.
81. Chan H-K, Young PM, Traini D, Coates M. Dry powder inhalers: challenges and goals for next generation therapies. Pharmaceutical Technology Europe 2007;19(4):19–24.
82. Damms B, Bains W. The Cost of Delivering Drugs without Needles. Nature Biotechnology 1995;13(12):1438–1440.
83. Patton JS, Bukar J, Nagarajan S. Inhaled insulin. Advanced Drug Delivery Reviews 1999;35(2–3):235–247.
84. Uchenna Agu R, Ikechukwu Ugwoke M, Armand M, Kinget R, Verbeke N. The lung as a route for systemic delivery of therapeutic proteins and peptides. Respiratory Research 2001;2(4):198–209.
85. Ward EM, Woodhouse A, Mather LE, Farr SJ, Okikawa JK, Lloyd P, et al. Morphine pharmacokinetics after pulmonary administration from a novel aerosol delivery system. Clinical Pharmacology and Therapeutics 1997;62(6):596–609.
86. French DL, Edwards DA, Niven RW. The influence of formulation on emission, deaggregation and deposition of dry powders for inhalation. Journal of Aerosol Science 1996;27(5):769–783.
87. Ticehurst MD, Basford PA, Dallman CI, Lukas TM, Marshall PV, Nichols G, et al. Characterisation of the influence of micronisation on the crystallinity and physical stability of revatropate hydrobromide. International Journal of Pharmaceutics 2000;193(2):247–259.
88. Young PM, Cocconi D, Colombo P, Bettini R, Price R, Steele DF, et al. Characterization of a surface modified dry powder inhalation carrier prepared by “particle smoothing”. Journal of Pharmacy and Pharmacology 2002;54(10):1339–1344.
89. Martonen TB. Mathematical model for the selective deposition of inhaled pharmaceuticals. 1993; 82(12): 1191–1199.
90. Labiris NR, Dolovich MB. Pulmonary drug delivery. Part I: physiological factors affecting therapeutic effectiveness of aerosolized medications. British Journal of Clinical Pharmacology 2003;56(6):588–599.
91. Stone KC, Mercer RR, Gehr P, Stockstill B, Crapo JD. Allometric relationship of cell numbers and size in the mammalian lung. American Journal of Respiratory Cell and Molecular Biology 1992;6:235–243.
92. Hickey AJ, Thompson DC. Physiology of the airways. In: Hickey AJ, editor. Pharmaceutica Inhalation Aerosol Technology: Marcel Dekker, Inc.; 1992. pp. 1–27.
93. Pritchard JN. The influence of lung deposition on clinical response. Journal of Aerosol Medicine—Deposition, Clearance and Effects in the Lung 2001;14:S19–S26.
94. Shoyele SA. Controlling the release of proteins/peptides via the pulmonary route. In: Jain KK, editor. Drug Delivery Systems. Totowa, NJ: Humana Press; 2008. pp. 141–148.
95. Schanker LS, Mitchell EW, Brown RA Jr., Species comparison of drug absorption from the lung after aerosol inhalation or intratracheal injection. Drug Metabolism and Disposition 1986;14(1):79–88.
96. Kim HK, Chung HJ, Park TG. Biodegradable polymeric microspheres with “open/closed” pores for sustained release of human growth hormone. Journal of Controlled Release 2006;112(2):167–174.
97. Wang J, Chua KM, Wang C-H. Stabilization and encapsulation of human immunoglobulin G into biodegradable microspheres. Journal of Colloid and Interface Science 2004;271(1):92–101.
98. Yamamoto H, Hoshina W, Kurashima H, Takeuchi H, Kawashima Y, Yokoyama T, et al. Engineering of poly(DL-lactic-co-glycolic acid) nanocomposite particles for dry powder inhalation dosage forms of insulin with the spray-fluidized bed granulating system. Advanced Powder Technology 2007;18(2):215–228.
99. Wang J, Ben-Jebria A, Edwards DA. Inhalation of estradiol for sustained systemic delivery. Journal of Aerosol Medicine 1999;12(1):27–36.
100. Vanbever R, Mintzes JD, Wang J, Nice J, Chen D, Batycky R, et al. Formulation and physical characterization of large porous particles for inhalation. Pharmaceutical Research 1999;16(11):1735–1742.
101. Ben-Jebria A, Chen D, Eskew ML, Vanbever R, Langer R, Edwards DA. Large porous particles for sustained protection from carbachol-induced bronchoconstriction in guinea pigs. Pharmaceutical Research 1999;16(4):555–561.
102. Koushik K, Kompella U. Preparation of large porous deslorelin-PLGA microparticles with reduced residual solvent and cellular uptake using a supercritical carbon dioxide process. Pharmaceutical Research 2004;21(3):524–535.
103. Yang Y, Bajaj N, Xu P, Ohn K, Tsifansky MD, Yeo Y. Development of highly porous large PLGA microparticles for pulmonary drug delivery. Biomaterials 2009;30(10):1947–1953.
104. Salama R, Hoe S, Chan H-K, Traini D, Young PM. Preparation and characterisation of controlled release co-spray dried drug-polymer microparticles for inhalation 1: influence of polymer concentration on physical and in vitro characteristics. European Journal of Pharmaceutics and Biopharmaceutics 2008;69(2):486–495.
105. Salama RO, Traini D, Chan H-K, Young PM. Preparation and characterisation of controlled release co-spray dried drug-polymer microparticles for inhalation 2: evaluation of in vitro release profiling methodologies for controlled release respiratory aerosols. European Journal of Pharmaceutics and Biopharmaceutics 2008;70(1):145–152.
106. Salama RO, Daniela T, Chan H-K, Sung A, Ammit AJ, Young PM. Preparation and evaluation of controlled release microparticles for respiratory protein therapy. Journal of Pharmaceutical Sciences 2009;98:2709–2717.
107. Liao YH, Brown MB, Jones SA, Nazir T, Martin GP. The effects of polyvinyl alcohol on the in vitro stability and delivery of spray-dried protein particles from surfactant-free HFA 134a-based pressurised metered dose inhalers. International Journal of Pharmaceutics 2005;304(1–2):29–39.
108. Dunne M, Corrigan OI, Ramtoola Z. Influence of particle size and dissolution conditions on the degradation properties of polylactide-co-glycolide particles. Biomaterials 2000;21(16):1659–1668.
109. Batycky RP, Hanes J, Langer R, Edwards DA. A theoretical model of erosion and macromolecular drug release from biodegrading microspheres. Journal of Pharmaceutical Sciences 1997;86(12):1464–1477.
110. Armstrong DJ, Elliott PN, Ford JL, Gadsdon D, McCarthy GP, Rostron C, et al. Poly-(D,L-lactic acid) microspheres incorporating histological dyes for intra-pulmonary histopathological investigations. Journal of Pharmacy and Pharmacology 1996;48(3):258–262.
111. Müller RH, Maaen S, Weyhers H, Specht F, Lucks JS. Cytotoxicity of magnetite-loaded polylactide, polylactide/glycolide particles and solid lipid nanoparticles. International Journal of Pharmaceutics 1996;138(1):85–94.
112. Sivadas N, O’Rourke D, Tobin A, Buckley V, Ramtoola Z, Kelly JG, et al. A comparative study of a range of polymeric microspheres as potential carriers for the inhalation of proteins. International Journal of Pharmaceutics 2008;358 (1–2):159–167.
113. Asada M, Takahashi H, Okamoto H, Tanino H, Danjo K. Theophylline particle design using chitosan by the spray drying. International Journal of Pharmaceutics 2004;270(1–2):167–174.
114. Huang YC, Yeh MK, Cheng SN, Chiang CH. The characteristics of betamethasone-loaded chitosan microparticles by spray-drying method. Journal of Microencapsulation 2003;20(4):459–472.
115. Sakagami M, Kinoshita W, Sakon K, Sato J-I, Makino Y. Mucoadhesive beclomethasone microspheres for powder inhalation: their pharmacokinetics and pharmacodynamics evaluation. Journal of Controlled Release 2002;80(1–3):207–218.
116. Smith J, Wood E, Dornish M. Effect of chitosan on epithelial cell tight junctions. Pharmaceutical Research 2004;21(1):43–49.
117. Yamamoto H, Kuno Y, Sugimoto S, Takeuchi H, Kawashima Y. Surface-modified PLGA nanosphere with chitosan improved pulmonary delivery of calcitonin by mucoadhesion and opening of the intercellular tight junctions. Journal of Controlled Release 2005;102(2):373–381.
118. Learoyd TP, Burrows JL, French E, Seville PC. Modified release of beclometasone dipropionate from chitosan-based spray-dried respirable powders. Powder Technology 2008;187(3):231–238.
119. Learoyd TP, Burrows JL, French E, Seville PC. Chitosan-based spray-dried respirable powders for sustained delivery of terbutaline sulfate. European Journal of Pharmaceutics and Biopharmaceutics 2008;68(2):224–234.
120. Grenha A, Remuñán-López C, Carvalho ELS, Seijo B. Microspheres containing lipid/chitosan nanoparticles complexes for pulmonary delivery of therapeutic proteins. European Journal of Pharmaceutics and Biopharmaceutics 2008;69(1):83–93.
121. Li FQ, Hu JH, Lu B, Yao H, Zhang WG. Ciprofloxacin-loaded bovine serum albumin microspheres: preparation and drug-release in vitro. Journal of Microencapsulation 2001;18(6):825–829.
122. Zeng XM, Martin GP, Marriott C. Preparation and in-vitro evaluation of tetrandrine-entrapped albumin microspheres as an inhaled drug-delivery system. European Journal of Pharmaceutical Sciences 1995;3(2):87–93.
123. Kwon MJ, Bae JH, Kim JJ, Na K, Lee ES. Long acting porous microparticle for pulmonary protein delivery. International Journal of Pharmaceutics 2007;333 (1–2):5–9.
124. Bosquillon C, Lombry C, Préat V, Vanbever R. Influence of formulation excipients and physical characteristics of inhalation dry powders on their aerosolization performance. Journal of Controlled Release 2001;70(3):329–339.
125. McConville JT, Patel N, Ditchburn N, Tobyn MJ, Staniforth JN, Woodcock P. Use of a novel modified TSI for the evaluation of controlled-release aerosol formulations. I. Drug Development and Industrial Pharmacy 2000;26(11):1191–1198.
126. Ho DH, Wang CY, Lin JR, Brown N, Newman RA, Krakoff IH. Polyethylene glycol-L-asparaginase and L-asparaginase studies in rabbits. Drug Metabolism and Disposition 1988;16(1):27–29.
127. Zalipsky S. Synthesis of an end-group functionalized polyethylene glycol-lipid conjugate for preparation of polymer-grafted liposomes. Bioconjugate Chemistry 1993;4(4):296–299.
128. Karathanasis E, Ayyagari AL, Bhavane R, Bellamkonda RV, Annapragada AV. Preparation of in vivo cleavable agglomerated liposomes suitable for modulated pulmonary drug delivery. Journal of Controlled Release 2005;103(1):159–175.
129. Garcia-Contreras L, Morçöl T, Bell SJD, Hickey AJ. Evaluation of novel particles as pulmonary delivery systems for insulin in rats. AAPS PharmSci 2003;5(2): Article 9.
130. Sharma A, Sharma US. Liposomes in drug delivery: progress and limitations. International Journal of Pharmaceutics 1997;154(2):123–140.
131. Allen TM. Liposomal drug formulations: rationale for development and what we can expect for the future. Drugs 1998;56(5):747–756.
132. Labiris NR, Dolovich MB. Pulmonary drug delivery. Part II: the role of inhalant delivery devices and drug formulations in therapeutic effectiveness of aerosolized medications. British Journal of Clinical Pharmacology 2003;56(6):600–612.
133. Thomas DA, Myers MA, Wichert B, Schreier H, Gonzalez-Rothi RJ. Acute effects of liposome aerosol inhalation on pulmonary function in healthy human volunteers. Chest 1991;99:1268–1270.
134. Alton E, Stern M, Farley R, Jaffe A, Chadwick SL, Phillips J, et al. Cationic lipid-mediated CFTR gene transfer to the lungs and nose of patients with cystic fibrosis: a double-blind placebo-controlled trial. Lancet 1999;353(9157):947–954.
135. Niven R, Pearlman R, Wedeking T, Mackeigan J, Noker P, Simpson-Herren L, et al. Biodistribution of radiolabeled lipid-DNA complexes and DNA in mice. Journal of Pharmaceutical Sciences 1998;87(11):1292–1299.
136. Smith PL. Peptide delivery via the pulmonary route: a valid approach for local and systemic delivery. Journal of Controlled Release 1997;46(1–2):99–106.
137. Wong JP, Yang HM, Blasetti KL, Schnell G, Conley J, Schofield LN. Liposome delivery of ciprofloxacin against intracellular Francisella tularensis infection. Journal of Controlled Release 2003;92(3):265–273.
138. Stark B, Andreae F, Mosgoeller W, Edetsberger M, Gaubitzer E, Koehler G, et al. Liposomal vasoactive intestinal peptide for lung application: protection from proteolytic degradation. European Journal of Pharmaceutics and Biopharmaceutics 2008;70(1):153–164.
139. Chono S, Tanino T, Seki T, Morimoto K. Efficient drug targeting to rat alveolar macrophages by pulmonary administration of ciprofloxacin incorporated into mannosylated liposomes for treatment of respiratory intracellular parasitic infections. Journal of Controlled Release 2008;127(1):50–58.
140. Bhavane R, Karathanasis E, Annapragada AV. Agglomerated vesicle technology: a new class of particles for controlled and modulated pulmonary drug delivery. Journal of Controlled Release 2003;93(1):15–28.
141. Cook RO, Pannu RK, Kellaway IW. Novel sustained release microspheres for pulmonary drug delivery. Journal of Controlled Release 2005;104(1):79–90.
142. Taylor KMG, Taylor G, Kellaway IW, Stevens J. The stability of liposomes to nebulisation. International Journal of Pharmaceutics 1990;58(1):57–61.
143. Darwis Y, Kellaway IW. Nebulisation of rehydrated freeze-dried beclomethasone dipropionate liposomes. International Journal of Pharmaceutics 2001;215 (1–2):113–121.
144. Kellaway IW, Farr SJ. Liposomes as drug delivery systems to the lung. Advanced Drug Delivery Reviews 1990;5(1–2):149–161.
145. Cortesi R, Esposito E, Luca G, Nastruzzi C. Production of lipospheres as carriers for bioactive compounds. Biomaterials 2002;23(11):2283–2294.
146. Jaspart S, Bertholet P, Piel G, Dogné J-M, Delattre L, Evrard B. Solid lipid microparticles as a sustained release system for pulmonary drug delivery. European Journal of Pharmaceutics and Biopharmaceutics 2007;65(1):47–56.
147. Sanna V, Kirschvink N, Gustin P, Gavini E, Roland I, Delattre L, et al. Preparation and in vivo toxicity study of solid lipid microparticles as carrier for pulmonary administration. AAPS PharmSciTech 2004;5(2): Article 27.
148. Evora C, Soriano I, Rogers RA, Shakesheff KM, Hanes J, Langer R. Relating the phagocytosis of microparticles by alveolar macrophages to surface chemistry: the effect of 1,2-dipalmitoylphosphatidylcholine. Journal of Controlled Release 1998;51(2–3):143–152.
149. Yamamoto A, Yamada K, Muramatsu H, Nishinaka A, Okumura S, Okada N, et al. Control of pulmonary absorption of water-soluble compounds by various viscous vehicles. International Journal of Pharmaceutics 2004;282(1–2):141–149.
150. Yamada K, Kamada N, Odomi M, Okada N, Nabe T, Fujita T, et al. Carrageenans can regulate the pulmonary absorption of antiasthmatic drugs and their retention in the rat lung tissues without any membrane damage. International Journal of Pharmaceutics 2005;293(1–2):63–72.
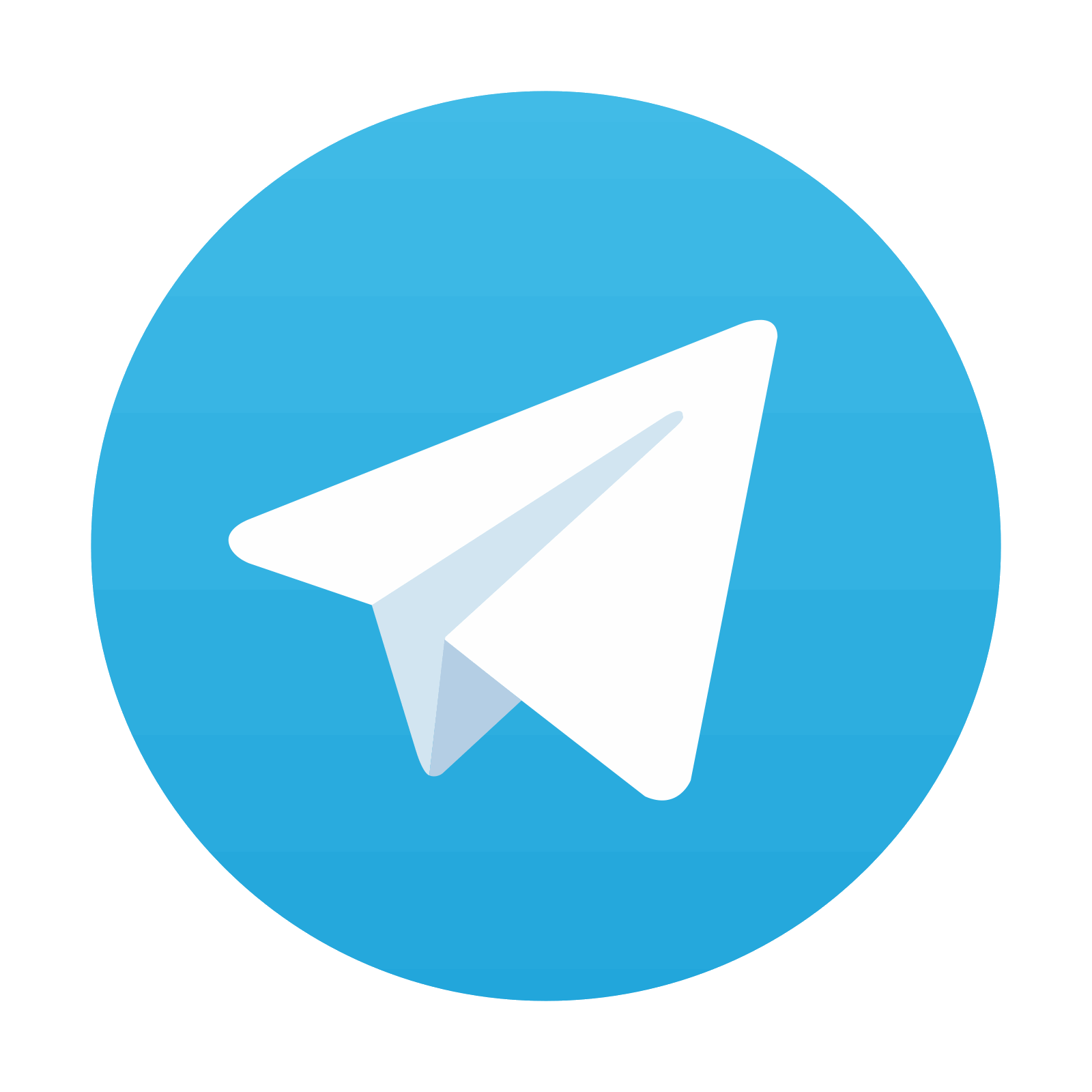
Stay updated, free articles. Join our Telegram channel
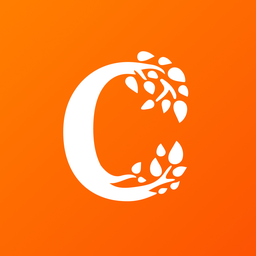
Full access? Get Clinical Tree
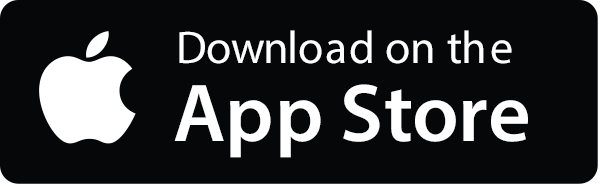
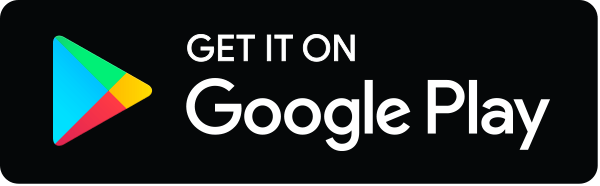