6.1 Introduction
Impactors are the official instruments for determining the particle size distribution of aerosols generated from medical inhalers and nebulizers. They directly measure the aerodynamic particle size, which affects how particles move in an airstream. At the same time, impactors provide the only means of separating the mass of active pharmaceutical agents into different size ranges, as well as other non-physiologically active components of the formulation. The aerosol clouds generated by inhalers typically have a single drug or a drug combination, lactose, or propellant as their main excipient, as well as adjuncts and other additives to improve the product performance.
Methods of aerosol particle sampling and sizing are executed by fluxing the aerosol through a series of stages of know dimensions. The aerosol is carried by the airflow inside the impactor and particles impinge upon a flat collecting surface, called plate. The orifice diameter of the stage entrance and the distance from the collecting flat surface are kept fixed. Particles with a high inertial force are unable to follow the streamlines and collide upon the first stages of the instrument. Smaller particles are able to remain airborne, following the streamlines through the successive impaction plates; with smaller and smaller sizes—and favorable aerodynamic properties—they impact upon lower and lower stages [1].
Impactors are used not to predict in vivo deposition but to carry out quality assessment. In vitro, the velocity of the particles increases from the first stage through successive stages; in vivo, velocity decreases from the throat to the alveoli.
Metered-dose inhalers (MDIs) consist of a solution or suspension formulation of drug in propellant and utilize a pressurized canister and valve to sample a precisely metered volume of the formulation. Dry powder inhalers (DPIs) on the other hand are made of finely divided solid drug particle clusters with or without particulate excipients. Pulmonary administration with a DPI requires an inhalation effort by the patient in order to deaggregate particle clusters. A higher inhalation flow rate produces a greater level of powder deaggregation, giving rise to individual particles for effective administration. In this case, the deliverable and respirable doses are dependent on the inhalation flow rate and tend to be inconsistent with each administration. This difficulty is further complicated by varying the aggregation degree of the powder in the inhaler during storage, under the influence of relative humidity. Recent DPIs exploit compressed air or battery-powered impellers to deaggregate the powder clusters without relying on patient inhalation flow rate.
In vitro aerodynamic assessment of the size and size distribution of particles, the fine particle fraction (FPF), and/or the mass that can reach the lungs provides a measure of inhaler consistency in drug delivery and in drug deposition pattern in the lungs [2]. Particles for inhalation have to be designed by tailoring their size and physicochemical properties. Their functionality is determined by aerodynamic assessment of the aerosol.
The currently accepted standardized test methods and apparatuses for inhaler quality assessment are documented in pharmacopoeias such as the European Pharmacopoeia and the United States Pharmacopoeia [3, 4]. Broadly, the aerodynamic profile of an aerosol cloud from an inhaler or nebulizer can be examined by means of a twin-stage impinger, Andersen Cascade Impactor (ACI), Marple–Miller impactor, Multi Stage Liquid Impinger (MSLI), or Next Generation Impactor (NGI).
6.2 Impactor/Impinger Design
Impactors are apparatus in which particles impact on a dry surface (i.e. a collecting plate). In impingers, particles deposit instead on a liquid surface. The general principle of inertial impaction is applied to both impactors and impingers.
Inertia impaction has been used to fractionate aerosolized particles in component sizes. It provides a direct measure of the aerodynamic size of particles. This is determined by collecting particles on an inertial collection apparatus, such as a multistage impactor. Dispersed in an airflow, particles will follow the direction of airflow until they lose inertia as a result of friction between particles and the surrounding medium. Then the particles are relaxed and moved into a different direction of flow. The “relaxation time” is the time required for a particle to adjust or relax its velocity to a new condition of drag forces. In an aerosol, small particles relax rapidly. They possess a lower level of momentum (the motion of a body, equal to the product of its mass and velocity). Small particles thus do not tend to be collected, while large particles impact on collection surfaces placed in the path of the original direction of airflow. Consequently, aerosolized particles are classified according to their differences in size.
Both impactors and impingers are built based on the concept of inertia impaction. The ACI and NGI (Figure 6.1) are the main instruments used in the development and quality characterization of inhalation products. They share many similar instrumental elements in their design and test protocol with the Marple–Miller impactor and MSLI. Typically, both impactors and impingers are a sequential assembly of an inhaler mouthpiece, induction port, series of collection stages, filter system, flow-control valve with absolute-pressure transducers, two-way solenoid valve, timer, and vacuum pump. Sometimes additional components are required, such as a preseparator, which is added to remove particles larger than 20 μm in the aerodynamic assessment of a DPI. The preseparator, placed between the induction port (throat) and the first impactor stage, is particularly useful in capturing the large particles of the lactose carrier when a dry powder blend formulation is being tested.
Figure 6.1 Cascade impactors, from left to right: multistage liquid impinger (MSLI), next-generation impactor (NGI), Andersen cascade impactor (ACI). Courtesy of Copley Scientific Limited, UK
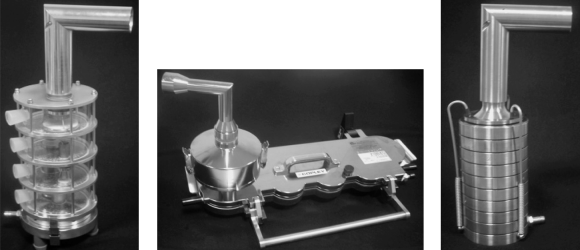
The main components of an impactor for the aerodynamic measurement of inhalation product are the induction port, impactor stages, and vacuum pump. The induction port (an aluminum or stainless-steel tube with a 90° bend) serves to simulate the mouth and throat of a patient, and to direct the airflow to align with the positions of connected impactor stages. The vacuum pump creates an airflow through the impactor stages, reproducing at the induction port the peak flow value of an inspiration act. The flow is kept constant for the duration of the test.
The impactor or impinger is made of a series of four to eight tightly sealed stages. Each impactor stage is characterized by a nozzle or a set of nozzles and an impaction plate [5]. Like the ACI, the most recently developed impactor (the NGI) consists of eight stages. The aerosol particles are accelerated through nozzles to an impaction plate. The larger particles will impact on to the plate and be collected, while smaller particles will travel with the airflow to impact on a later plate. The impactor stages collect aerosolized particles into various fractions of different particle sizes. The final integral part of an impactor or impinger is a filter or microorifice collector (MOC), which collects the nonimpacted particles from the airflow. Particles <0.1 μm deposit by Brownian diffusion: an irregular wiggling motion in still air from a region of higher concentration to a region of lower concentration. Brownian diffusion, interception, charge, sedimentation, and inertia impaction are all deposition mechanisms which contribute together to the deposition of particles.
6.3 Aerodynamic Assessment
Aerodynamic assessment is performed through a series of experiments and successive calculations aimed at determining the aerodynamic size distribution of aerosol particles. The aerodynamic diameter is the characteristic parameter used to express a particle’s ability to follow an airstream. It assesses in vitro the respirability of a product. The aerodynamic assessment is performed using impactors, by measuring the number of particles deposited on the various stages. An impactor stage has a “cut-off size”: all particles above a certain size are collected, while all particles below that size pass through. This sequential separation has the effect of classifying the particles into groups according to their aerodynamic diameter. From the amount of sample collected at each stage, the fraction of the total mass in each aerodynamic size range can be determined using the procedure described in Section 6.8.
The aerodynamic diameter is a characteristic equivalent spherical diameter derived using the Stokes equivalent diameter.
- The Stokes diameter (dSt) is the diameter of a sphere with the same density and settling velocity as the particle.
- The aerodynamic diameter (dae) is the diameter of a sphere of unit density that has the same settling velocity as the particle.
Stokes’ law describes the particle sedimentation under gravity in a fluid. The application of Stokes’ law allows the determination of the settling velocity of aerosol particles undergoing gravitational settling in air. The terminal settling velocity, Vts, of a particle increases rapidly with particle size, being proportional to the square of the particle diameter. Vts is calculated by the formula:
(6.1)
where ρ is the density of spherical particles, g is the acceleration due to gravity, and η is the viscosity of the fluid where the particle settles. Hence the relation which links the two diameters is as follows:
(6.2)
Therefore, the aerodynamic diameter is the diameter of a hypothetical spherical particle of unit density (i.e. ρ0 = 1.00 g/cm3) that settles in air at the same falling velocity as the real particle. A correction factor, called the dynamic shape factor (χ) (included in dSt), is introduced into the relationship between dae and dv (equivalent volume diameter) in order to consider the effect of shape on particle motion. The shape factor is equal to 1 for a sphere and—except for certain streamlined shapes—the dynamic factor is greater than 1.0. The shape factor introduction allows the aerodynamic diameter of an aerosol to be calculated using the equivalent volume diameter by the formula:
(6.3)
where dv is the spherical equivalent volume diameter and χ is the dynamic shape factor. Owing to deformation by airflow stresses, the droplets of MDI product are not completely spherical. Inferring from this equation, the nonspherical particles are likely to exhibit a smaller aerodynamic diameter.
Principally, the aerodynamic diameter of an aerosol is dependent on particle properties such as geometric size, shape, density, and surface morphology. Porous particles will have a smaller aerodynamic diameter than dense particles. The aerodynamic property of liquid aerosol experiences a greater propensity of complication than that of DPI products, since solvent evaporation, phase separation, and the influences of additives greatly modify the properties of aerosol particles.
The particle size distribution of an aerosol typically follows a log-normal distribution pattern. It can be characterized by mass median size, which denotes diameter where 50% of the aerosolized particles are larger or smaller than the stated size. The distribution of particle size is described by geometric standard deviation (GSD). Using an impactor or impinger, which separates particles with an airflow and quantifies drug mass against their sizes, the size of an aerosol is termed the “mass median aerodynamic diameter” (MMAD). The computation of the MMAD of an aerosol proceeds by plotting on a log-probability scale the cumulative percentage of drug mass retained on each stage with the smaller cut-off diameter versus the logarithm of the cut-off diameter of the stage. The linearity of the relationship between the cumulative percentage of drug mass and log cut-off diameter is determined by least-squares regression. MMAD is the particle size below which 50% of the population falls and is often denoted as dae50. The GSD of aerosol size distribution, which expresses the particle distribution around the MMAD value, can be calculated from the slope of the same plot, as illustrated in Section 6.8 [4].
The aerodynamic diameter depends on the airflow around the particle, which is expressed through the Reynolds number (Re):
(6.4)
where ρ is the density of the fluid flow, V is the relative velocity between the fluid and an object such as a particle, d is the diameter of the particle, and η is the viscosity of the fluid in which particle settles.
Care should be taken to note that the density is that of the gas, not the aerosol particle—a frequent source of confusion in the application of Re to aerosol particles.
Re is a dimensionless number that indicates whether the flow of a fluid (liquid or gas) is absolutely steady (laminar flow) or steady on average but with small unsteady changes (turbulent flow).
The human respiratory system between the trachea and the terminal bronchioles exhibits a laminar airflow with an Re of 0.01–2. The airflow through the impactor has an Re of 0.1–20. The Stokes flow regime (laminar flow) depicts an aerodynamic diameter related to particle deposition by sedimentation and Brownian diffusion. The use of Stokes flow in the aerodynamic assessment of aerosol at high airflow rates is envisaged to lead to systematic errors. At Re > 0.1, inertial impaction represents the major deposition mechanism at upper airways.
The performance of a product for inhalation depends on both the aerodynamic and the geometric particle size distribution of the aerosol. The geometric particle size and size distribution determine the interparticle interactions, delivered dose and dose uniformity, drug dissolution rate, and cellular particle uptake. The aerodynamic particle size distribution and MMAD of aerosolized particles govern the respirable dose and particle deposition pattern in the lungs. Aerosol particles are deposited in the lungs by three main mechanisms: impaction, sedimentation, and Brownian diffusion. Particles with an MMAD ranging between 1 and 5 μm are known to deposit in bronchial and alveolar regions and demonstrate pulmonary penetration. Smaller particles within this size range exhibit a greater propensity of lung penetration. They reach distal airways and have more peripheral deposition. They are suitable for delivery of anti-inflammatory drugs and drugs with systemic effects which have to be absorbed in the alveolar region, such as peptides and proteins. Nonetheless, larger particles are more efficacious where the treatment of asthma is concerned, since the deposition in this case is required in the upper airways. The depositional target of particles of a size of 5 μm is the upper lung airway, where they give rise to a direct bronchodilation effect.
6.4 Inertial Impaction and Cut-Off Diameter
Particle Stokes number is a dimensionless parameter which governs the efficiency of particle impact on a collection plate, defined for an impactor as the ratio of particle stopping distance at the average nozzle exit velocity (U) to the nozzle radius. It is calculated according to the following expression:
(6.5)
where U0 is the average fluid velocity to the nozzle, ρ is the particle density, d is the particle diameter, Cc is the Cunningham slip correction factor, η is the dynamic viscosity of air, and Dn is the nozzle diameter.
The Cunningam correction factor is a factor applied to Stokes’ law for particles less than 1 μm in diameter which settle faster than predicted by Stokes’ law because there is a “slip” at their surface.
The equation assumes that all particles are spherical, that their Re is much less than 1 (<0.1), and that their density is greater than air density. A particle will impact on to a plate if its Stokes number is larger than approximately unity, which translates to a need for a longer relaxation time.
The collection efficiency is the fraction of particles passing through impactor nozzles which are retained on impaction plates. All particles larger than an impactor stage’s cut-off diameter will impact on that stage and all particles smaller than the cut-off diameter will follow the airflow through to successive impactor stages. This cuts the distribution of mass into a series of size ranges called “dimensional classes.”
The cut-off diameters of impactor stages must be regularly calibrated, as nozzles and collection plates can corrode with time and usage [6].
In testing a DPI using an impactor or impinger, an airflow rate between 28 and 100 L/min may be used, since DPIs have dissimilar powder flow resistances and dispersion efficiencies [7]. Using a different airflow rate Q from Qn (60 L/min) in association with the previously calibrated cut-off diameter, the new cut-off diameter of each stage at Q can be calculated using a preestablished equation:
(6.6)
where D50Q is the cut-off diameter at airflow rate Q (60 L/min) and n is the nominal cut-off value determined by Qn.
In the case of NGIs, a similar equation may be used to compute the cut-off diameter of impactor stages subjected to different airflow rates, except that the exponent value (x) varies with the impactor stage:
(6.7)
Given an airflow rate Q higher than Qn, the cut-off diameter of an impactor stage becomes smaller. The drug mass distributed across impactor stages is expected to exhibit a smaller aerodynamic size. In the calibration of impactor stages and testing of an inhalation product, the quantum of airflow rate will be specified. Each impactor stage must be calibrated and/or its cut-off diameter characterized at the airflow rate employed in aerodynamic testing of the inhalation product.
6.5 Pharmacopoeial Procedure
The aerodynamic assessment of inhalation product begins with the assembly of the impactor or impinger. The volumetric flow rate at the entrance to the induction port must be accurately set before every determination of aerodynamic particle size distribution. The airflow rate used in testing MDIs is kept at 28–30 L/min, but it can vary between 28 and 100 L/min in the testing of DPls. For the vast majority of DPls, it is unnecessary to coordinate breathing with the activation, since the patient simply inhales deeply to access the drug. It follows that the efficacy of the administration is dependent on the strength and duration of the patient’s inspiration. Furthermore, different inhalers provide varying degrees of resistance to inhalation. The in vitro assessment approximates the in vivo inspiration conditions, and the strength and the duration of the patient’s inspiration in particular are simulated by, respectively, the flow rate used and the time it takes the flow to pass through the device. In DPIs, the United States Pharmacopoeia states that the airflow rate corresponds to a pressure drop of 4 kPa over the device, and a duration consistent with the withdrawal of 4 L of air from the mouthpiece of inhaler should be employed. These parameters are described in the section “General Control Equipment” in the United States Pharmacopoeia [4] and the section “Experimental Set Up” in the European Pharmacopeia [3, 8].
6.5.1 The Duration of In Vivo Inspiration Needed for 4 L
The volume of air drawn through an inhaler during testing is kept at 4 L, as this volume represents the normal inspiratory capacity of an average-sized adult male weighing approximately 70 kg. A pressure drop of 4 kPa over the DPI broadly represents the pressure drop generated by patients using the device [9].
Prior to testing of a DPI, the intended airflow rate is set. The duration is simulated through the use of a two-way switching valve connected to a vacuum pump. The operation of the solenoid valve and hence the duration of the cycle is controlled by means of a timer. The valve is connected to both the impactor and the vacuum pump. In pre-test mode, the solenoid valve is in the closed position, such that no flow passes through the test apparatus.
On initiation of the test, the solenoid valve switches, so that flow now passes through the test apparatus and hence the inhaler under test. On expiration of the pre-set time, the solenoid closes again and the “inhalation” cycle is complete.
By using the timer to control how long the solenoid valve is open for, it is possible to control the volume of air drawn through the inhaler to the 4 L specified. It is also possible to establish for how many seconds (T) the valve must be in open mode in order to obtain 4 L of air (±5%) at the test flow rate QOUT:
(6.8)
For example, if the flow rate Q is 100 L/min, the timer should be set to 2.4 seconds. If the flow rate is 60 L/min, the timer should be set for 4 seconds. And if the flow rate is 30 L/min, the timer should be set for 8 seconds. The inspiration time is important for the DPls.
Q depends on the characteristics of the device used.
6.5.2 Setting Flow Rate, Q, to Give A Pressure Drop of 4 kPa
To set the correct flow rate to be used in the test, it is first necessary to establish the flow rate that produces a pressure drop comparable with that found in vivo when using the particular inhaler under study. The flow rate to be used is the flow rate that produces a pressure drop through the inhaler compatible with the resistance of the device. Both the European and the United States Pharmacopoeia suggest a pressure drop over the inhaler of 4 kPa is broadly representative of the pressure drop generated by patients using powder inhalers during inhalation [3, 4]. The pressure drop created by the air drawn through an inhaler can be measured directly by comparing the absolute pressure downstream of the inhaler mouthpiece to the atmospheric pressure.
Using a flow-control valve, it is now possible to adjust the flow rate from the vacuum pump to produce the established pressure drop of 4 kPa. By replacing the inhaler with a flow meter, it is then possible to measure the flow rate required to produce this pressure drop. This flow rate should be used for determination of both the delivered dose and the particle size. The only exception to this criterion is that if the flow rate required to produce a 4 kPa pressure drop is greater than 100 L/min, 100 L/min should be used.
6.5.3 Flow-Rate Stability
Having set the required parameters to control the peak and the duration of the simulated inspiration, there is one final variable which needs to be considered: the flow-rate stability. The flow rate can be assumed to be stable if the ratio P3/P2 is ≤0.5, where P2 represents the pressure at a point nearer the impactor or impinger and P3 represents the pressure at a point nearer the vacuum pump. This parameter is important to ensuring that the flow rate achieved through the inhaler is unaffected by pump fluctuations and minor changes in flow resistance upstream of the flow-control valve.
6.6 Cascade Impactor: General Set-Up and Operation
Proper set-up and operation of an impactor is critical for correct particle size measurement. A test failure can arise if the system is not properly assembled or the airflow is not checked prior to running the analysis. Errors can come from the collection plates or filter being wrongly located in the ACI, from air leakage into the apparatus, or from an improper alignment between inhaler mouthpiece and induction port [10]. During testing of the inhalation product, the inhaler is first primed or loaded and inserted in axis with the entry to the induction port (Figure 6.2). Each device has to fit properly with the induction port, through the use of a house-made rubber adaptor.
Figure 6.2 Alignment between the RS01 DPI inhaler (Plastiape, IT), mouthpiece, and induction port of the NGI. RS01 DPI – Plastiape. Courtesy of Plastiape IT, Courtesy of Copley Scientific Limited, UK
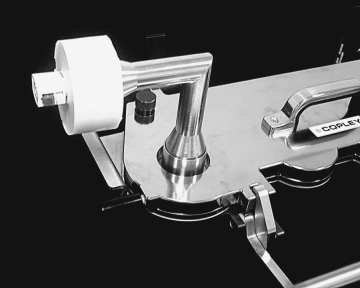
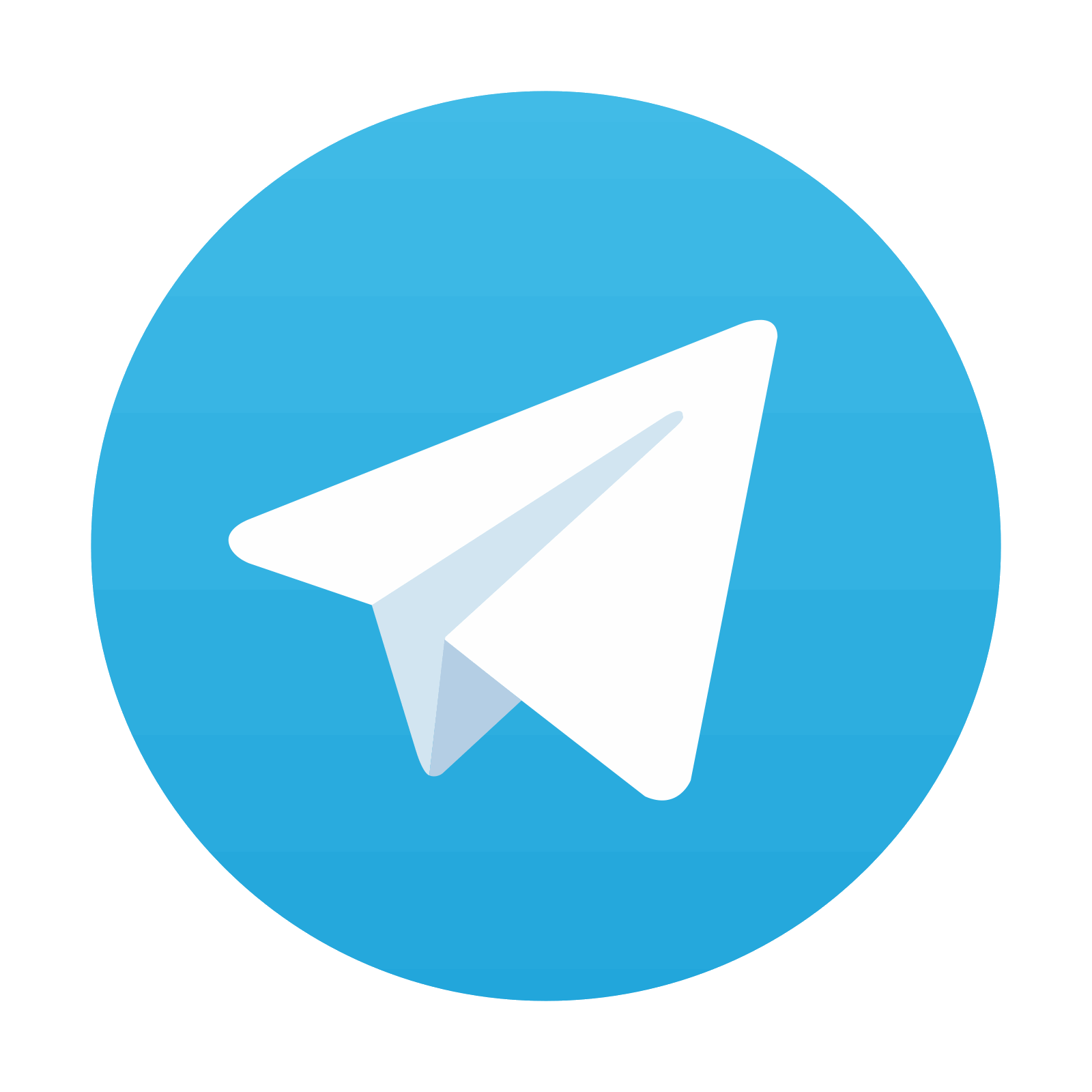
Stay updated, free articles. Join our Telegram channel
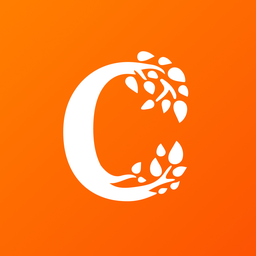
Full access? Get Clinical Tree
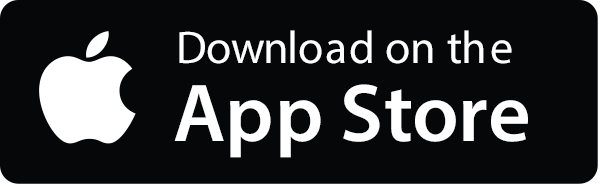
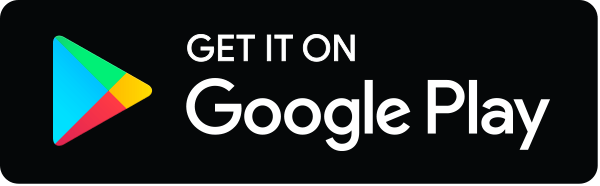