16 David B. Mayfield, Ari S. Lewis, Lisa A. Bailey, and Barbara D. Beck Investigations into the effects of metals on the human body have been of interest to physicians, scientists, and in some cases, those of a criminal bent, for millennia. The ancient Romans, Egyptians, Chinese, and other civilizations were aware of the effects of multiple metals, such as lead, antimony and arsenic. Metals were understood to elicit various toxicological reactions, particularly from high-level acute exposures eliciting readily apparent symptoms. Metals were also perceived to have various therapeutic abilities, such as curing coughs and asthma (arsenic), treating hangovers and other overindulgences (antimony), and curing tuberculolis (lead). The more sinister side of metals is another important part of their history. This is especially relevant with arsenic, a metal (actually a metalloid) with a particularly notorious history. Arsenic was desirable as a homicidal tool because the symptoms of acute arsenic poisoning are relatively general, making determination of cause of death difficult. However, the development of the Marsh test in the nineteenth century for detection of arsenic in body fluids reduced, but did not eliminate, its use for this purpose. As recently as 2003, arsenic was used intentionally in a poisoning incident in the United States in the state of Maine. The toxicity of metals remains a robust area for scientific inquiry today. The awareness of exposure sources has expanded over time and includes pharmaceutical products, environmental media, consumer products, and workplace. Interest in types of metals has also expanded to include a broad range, some of which, such as the rare earth elements (REEs), that is, the lanthanides, have only been studied recently. New effects of certain metals already relatively well studied are being identified, for example, gastrointestinal (GI) tumors in rodents ingesting hexavalent chromium in drinking water. And methodologies for investigating metals have advanced well beyond descriptive studies of clinical effects, reflecting greater understanding of toxicological mechanisms at the cellular and molecular levels. Consider, for example, the use of biomarkers to evaluate exposures to and effects of metals. In this chapter, we Metals are naturally occurring elements that are present throughout the environment. Many metals are essential nutrients and are required at some minimum level for good health; therefore, the distinction must be made between necessary minimal exposure and toxic overexposure. Organisms, including humans, have evolved detoxifying mechanisms to cope with potentially harmful levels of both essential and nonessential metals since exposure to metals occurs regularly in the environment. It is when the frequency, magnitude, or duration of exposure exceeds the detoxification capacity that metal toxicity may become a concern. Metals and metalloids are typically found in a solid state at room temperature, except for elemental mercury, which exists in the liquid phase. Metals can transform from solid to liquid or liquid to gas depending on their respective melting and boiling points. Due to their physical state, exposure to metals via inhalation primarily occurs in particulate form or as a dust or fume. Exceptions are elements that occur in gaseous form at certain temperatures (e.g., mercury vapor, arsine gas (AsH3), and stibine (SbH3)). Metals are also common in foods, soils, and tissues complexed with organic matter or in dissolved form complexed with ionic compounds (e.g., chlorides or sulfates). These metal complexes account for metal ingestion exposures via water, soil, and diet. Metals, like all elements, cannot be broken down or degraded and are therefore persistent in the environment. They can, however, form compounds with other elements and chemicals. The primary feature that distinguishes metals from nonmetals is their ability to readily lose electrons in solution and form positively charged ions (cations). The cations can form strong ionic bonds (complexes) with nonmetals. While some important metals may be found in their elemental state (e.g., mercury) in the environment, most metals are found in a wide variety of complexes with other substances. The interactions of metal cations with their surroundings drive both their mobility in the environment and their biological effects. The various metal complexes, even with the same metal cation, may differ dramatically in their chemical and toxicological properties (e.g., elemental mercury vs. methyl mercury). While humans possess effective means for detoxifying and excreting metals at exposure levels normally encountered in the environment, some human activities lead to increased exposure to metals. In occupational situations, protection against exposure to metals is controlled through the use of improved work practices and protective equipment or by simply reducing exposure. Exposure to metals in the general population has also increased. Mining, processing, commerce, and waste disposal have all increased the movement of metals in the environment, both directly and indirectly, and contribute to higher background levels of exposure in general. In this chapter, properties and potential effects of both occupational and environmental exposure to metals are discussed. Metals are naturally present in the earth’s crust, and thus human exposure from natural sources is inevitable. Metals are found in a range of concentrations in soils, sediments, surface and ground waters, and air. Table 16.1 lists the typical levels of some metals found in the environment. It is important to remember that these levels may vary considerably due to geologic characteristics, land use (industrial vs. rural vs. urban), and the effects of human activities (e.g., mining operations and hazardous waste facilities). Thus, the levels reported in Table 16.1 are only ranges for comparative purposes and are not expected to represent background levels in all areas. Table 16.1 Typical Environmental Concentrations of Metals Soil and groundwater concentrations for the 10th and 90th percentile concentrations are given. Food and air concentrations are given as typical ranges, as defined by the ATSDR, and singular estimates of average intakes for adults. For lead, the air and food sources are highly dependent on local conditions, so the ATSDR did not present a typical range.nm, nanometer. Metals move through the environment as part of natural biogeochemical pathways: dissolved from rocks; transported in soils, water, and air; taken up from soil and water by plants; passed up the food chain; and released back to the environment through excretion and decay of dead organisms and often going through various transformations in the process. Metals may form a variety of compounds by combining with available negatively charged groups, such as chlorides, sulfates, nitrates, carbonates, and acetates. The metal complexes can differ dramatically from the uncomplexed metal in their solubility and their absorption by and distribution within living organisms. Therefore, the toxicological properties of metals and their complexes can vary significantly. Speciation is the primary characteristic that determines a metal’s potential for exposure and its resulting toxicity. A metal may exist in its elemental, uncharged, form and this state is often designated by a “0” representing “zero charge” (i.e., Hg(0) or Hg0 for elemental mercury). This is typically the form of objects that we think of as being metallic. However, a property common to all metals is the tendency to ionize in solution, giving up one or more electrons to become a cation or a compound with a net positive charge (e.g., for cadmium, Cd → Cd2+ + 2e−). Metals may exist in a variety of ionization states, or valences, that differ in the number of missing electrons and therefore exhibit various net positive charges. The standard designation for the first ionization state of many metals is the name of the metal with an ous suffix, such as mercurous ion for Hg+ and cuprous ion for Cu+. The next level of ionization is sometimes designated with an ic suffix, such as mercuric for Hg2+ and cupric for Cu2+. Ionization is not a uniform process across all metal types, and a metal may give up two electrons in its first ionization state (e.g., for tin, the stannous ion is Sn2+) or even three electrons (e.g., trivalent chromium is Cr3+ or arsenite is As3+). Other ionization states may exist for some metals, but usually the number of states most commonly encountered under normal conditions is limited to two or three. The degree to which this process is carried out will, in turn, affect various metal characteristics, including its toxicity. Metal speciation is heavily dependent on physical parameters such as pH (the concentration of H+ ions), oxidizing or reducing conditions (often measured as reduction potential (Eh) or oxidizing–reducing potential), and temperature. The presence of other charged particles that can bind with the metals (known as ligands) in the air, soil, or water is another strong determinant if the metal will remain as a free cation or form a complex. These parameters determine how and at what rates metals may move through the environment, which must be considered when assessing the primary routes of exposure and potential concentrations during exposure. Metal cations are typically more mobile than their elemental forms, but it is difficult to generalize how other external factors influence metal mobility since a single factor might increase the mobility of one metal while dramatically reducing that of another. For example, a common ligand in groundwater, sulfate, enhances the solubility (and therefore mobility) of cadmium and zinc, while barium sulfate is nearly insoluble and is not readily transported. An example of the degree to which these chemical and physical factors affect human exposure to metals can be illustrated by copper. Copper has an especially high affinity for organic ligands. In aquatic systems, copper is ionized and readily binds to organic matter, settles out of the water column, and is unavailable to most organisms. Likewise, copper cations bind to organic matter in soil and tend to accumulate in the upper layers of soil when applied at the surface, as would occur from deposition from air or as runoff from roadways. This surface accumulation decreases the likelihood of oral exposure to copper through contaminated groundwater but increases the likelihood of exposure through soil contact. Chemical and physical attributes (e.g., chemical species, exposure matrix, and particle size) of metals will also affect absorption into the bloodstream. That is, a metal’s bioavailability depends on both the innate properties of that metal as well as how it interacts with environmental media. For example, arsenic is almost 100% bioavailable in water, but in a soil matrix, absorption into the body will be significantly less. The extent of bioavailability is often limited by binding to ligands in the soil and will be influenced by a variety of soil characteristics including the iron and organic matter content as well as the pH. These factors have the potential to reduce the oral bioavailability of arsenic to 50% or less. In the case of metal exposure through inhalation, smaller particles typically result in greater bioavailablity, in part because smaller particles will penetrate deeper into the respiratory tract. In addition to environmental factors, the bioavailability of a metal can also be influenced by host factors, such as gender, age, nutritional status, and other health conditions. Speciation of metals and conversion from one form to another in the environment may also occur as a result of biological action, termed biotransformation. The metabolic processes of many microorganisms can transform metal compounds to more or less toxicologically important forms. In particular, the methylation of metals often results in complexes that are more easily absorbed and therefore potentially more toxic. For example, in contaminated aquatic systems, the transformation of ionic mercury to methyl mercury and to dimethyl mercury is driven by anaerobic microorganisms. Likewise, inorganic lead may be biotransformed by some bacteria to tetraethyl lead, an organic compound that is more easily transported across biological membranes. Biological processes can also work to make metals less available, such as when metals are absorbed from the soil and groundwater by plants and become incorporated into their tissues. For example, the methylation of inorganic arsenic reduces toxicity because methylated forms of arsenic are poorly absorbed into human cells. In addition to natural biogeochemical cycles, humans play a large role in the mobilization, transformation, and transportation of metals in the environment. Industrial activities, such as mining and manufacturing, often concentrate metals from natural minerals and this increases the potential for human exposure. Some of the concentrated metals may be incorporated into new products and become widely distributed, while others are simply discarded as waste. Human activities can also indirectly alter natural biogeochemical cycles and change how metals move through the environment. A potential by-product of mining is the release of high levels of acid, which mobilizes naturally present metals, into local streams and is known as acid mine drainage. Occupational exposures typically occur during the initial processing of minerals or in the manufacturing of new products (e.g., zinc used for galvanizing steel and lead released during the smelting process). Exposure assessment for the general population must consider the life cycle of a product from its origin in industry, the patterns of use, and ultimately the disposal of both manufacturing and product wastes. Exposures to high levels of metals are usually of greatest concern for workers in industries where metals are commonly used. These include mining, processing and smelting, manufacturing, and waste disposal operations. The metals of concern in occupational settings are dependent on the specific industry. For instance, sheet metal workers may be exposed to copper and aluminum dust particles, while lead ore smelting workers may be exposed to releases of lead, arsenic, and cadmium. Although workers are often exposed to higher metal concentrations than the general public, health and safety regulations are designed to maintain exposure at safe levels through improved work practices and personal protective equipment, when necessary. Because workplace exposure is often confined to a specific site with a specific population at risk, routine monitoring of exposure can be performed and corrective action can be taken when necessary to maintain safe limits. People living near industrial areas, waste sites, mining operations, or smelters may be exposed to higher-than-background levels of metals in air, drinking water, and soil. Industrial contamination of local environments has resulted from accidental releases as well as improper management practices. One of the first documented cases of widespread illness from incorrect waste disposal occurred in Japan and underscored the dangers of mercury. Beginning in the 1930s, industrial wastes containing methyl mercury were discharged into Minamata Bay, where it bioaccumulated in fish and shellfish. By the late 1950s, severe neurological impairment, sometimes leading to death, was observed in both adults and children and was later linked to the consumption of mercury in seafood. The neurological symptoms described there later became known as Minamata disease. Increased exposure to metals can also occur through the normal use of products. The use of lead in gasoline and paints resulted in perhaps the most well-known example of increased metal exposure to the general population. While lead was added to both products to improve special characteristics, this had the unintended consequence of increasing the amount of lead in dust and soils to levels far above background concentrations in urban areas. The exposures to airborne lead particles from leaded gasoline as well as dust and soil at homes with lead paint contributed to an overall increase on the lead body burden in the general population and resulted in significantly higher blood lead levels (BLLs) in young children. The discontinuance of lead as an additive has dramatically decreased the impact of these exposure routes, and BLLs have decreased throughout the population. In some areas, naturally occurring metals found in soils, sediments, surface and ground waters, and air may be a significant source of exposure. For example, naturally occurring sources of uranium have caused high levels of radioactivity in groundwater in the Floridan aquifer system in southern Georgia. The level of radioactivity, above acceptable limits for drinking water, has resulted in the need for alternative drinking water sources. Some areas of the United States and other parts of the world have groundwater with elevated arsenic of geologic origin. China, Bangladesh, and Taiwan are among the areas of the world with the highest levels of naturally occurring arsenic in groundwater. Arsenic-containing water in areas of these countries can pose a serious public health concern, increasing the risk of many types of cancer and noncancer effects (such as skin lesions, cardiovascular disease, and respiratory illness). Although not a public health concern, another naturally occurring metal with significant human exposures is aluminum. Aluminum enters the environment through the weathering of rocks and minerals, and, because of its abundance in the earth’s crust, natural weathering processes far exceed releases into air, water, and land from anthropogenic sources. Aluminum is also found naturally in many foods. For a metal to exert a toxic effect on a human or other organism, there must be exposure and it must gain entry into the body. There are three main routes of absorption: inhalation, oral, and dermal. The significance of the route is metal-specific. For instance, copper is highly soluble in water so that ingestion (oral exposure) is a common pathway of exposure. Ingestion is also an important exposure pathway of mercury, often through the consumption of fish or other animals that bioaccumulate mercury to a high level in their tissues. However, the elemental form of mercury has a high vapor pressure so that inhalation exposure also may be significant in certain situations. Dermal exposure to metals may cause local effects, but it is rarely a significant consideration from an absorption perspective. Absorption through dermal barriers occurs primarily through passive diffusion, which is influenced by molecular weight, lipid solubility, temperature, pH of the substance, concentration, and integrity of the skin. Contact dermatitis and allergic skin reactions may occur in some individuals from dermal exposure to certain metals (e.g., beryllium, nickel, chromium, and cobalt). The route of absorption may also influence the subsequent distribution of the metal within the body and, thus, may affect its metabolism, potential toxic effects, and excretion. For example, while elemental mercury is effectively absorbed via inhalation into the bloodstream where it can cross the blood–brain barrier and cause neurological effects, elemental mercury is not well absorbed through the oral or dermal route and is essentially nontoxic via these pathways. In contrast, organic forms of mercury are well absorbed through all routes and have even resulted in death via dermal exposure. Once metals have entered the bloodstream, they are available for distribution throughout the body. The rate of distribution to organ tissues is determined by blood flow to the particular organ. The eventual distribution of a metal compound is largely dependent on the ability of the compound to pass through cell membranes, coupled with its affinity for binding sites. Metals are often concentrated in a specific tissue or organ (e.g., lead in bone and cadmium in liver), and their metabolism within the body usually involves binding to proteins, such as enzymes, or changes in their speciation. Metals also may bind to other substrates and alter the bioavailability of important cell constituents. The simplest method of excreting metals is to exclude them before they achieve significant absorption. For instance, inhaled metal vapors may be immediately expired during subsequent breaths, while inhaled particles may be trapped in the mucous and expelled by the cough reflex. Vomiting is a common reflex in response to orally ingested toxicants, including metals. Metals may also be excreted in the sweat and saliva or incorporated into growing hair and fingernails (through complexes with sulfhydryl groups). The body’s attempt to excrete excess mercury and lead in the saliva often results in visible “lead lines” along the gums. Urine and fecal excretion, however, remains the primary route of excretion for most ingested metals and are also important following inhalation and dermal exposure. Usually, excretion consists of a combination of these pathways, which may differ according to the route of exposure and the speciation of the metal. Inhaled elemental mercury vapor, for instance, is excreted in the urine, feces, and breath, while ingested elemental mercury is primarily excreted in the feces. Methyl mercury is excreted very slowly, primarily in the feces after transformation to inorganic forms of mercury. Additionally, a common means by which the body defends itself against metal poisoning is by producing nonenzymatic proteins, which bind to and inactivate the metal, then transport it out of the body via the excretory system. Lipoproteins located within renal lysosomes appear to function in this way, thus serving as some protection for the kidneys, which are particularly vulnerable to metal damage. Metallothioneins (a class of sulfur-containing, metal-binding proteins that facilitate metal excretion) display a substantially increased expression in response to cadmium, mercury, zinc, and several other metals. A number of antidotes to metal poisoning have been developed on the basis of knowledge of natural detoxification mechanisms. Chelation therapy involves systematic treatment of the patient with a chelator, defined as a molecule with several electronegative groups able to form coordinate covalent bonds with metal cations and thus render the metal unavailable and inactive. An example of a chelator is ethylenediaminetetraacetic acid (EDTA), a flexible molecule with four binding sites capable of nonspecifically binding metal ions and escorting them to the kidney for excretion. Calcium EDTA is particularly effective against lead poisoning. British anti-Lewisite (dimercaprol; BAL) is another chelator that was developed during World War II as an antidote against a form of arsenic gas (British Lewisite) and has since found application in therapy for chromium, nickel, cobalt, lead, and inorganic mercury poisoning. Dimercaptosuccinic acid (DMSA) is a water-soluble chelating agent similar to BAL, which is used to treat heavy-metal poisoning. DMSA has fewer side effects than BAL. Although there are many benefits to chelation therapy, it must be used with discretion since chelators have been shown to result in redistribution of toxic metals, hepatotoxicity and nephrotoxicity, headache, nausea, and increased blood pressure. Chelation therapies are nonspecific in nature and, in addition to the target metal (e.g., arsenic and lead), can bind to other essential trace metals (e.g., calcium, copper, and zinc), leading to deficiency of these essential metals and unintended health effects if their use is not monitored appropriately. Some chelators have been shown to increase the biologic activity and toxicity of the metal. For example, EDTA is not able to shield the surface of the iron ion (Fe3+) but instead forms an open basket–like complex, resulting in increased catalytic capacity for Fe3+ to generate oxidative stress. BAL also has been shown to worsen the effects of selenium and methyl mercury intoxication. Physiologically based pharmacokinetic (PBPK) models use empirically based mathematical descriptions of uptake and distribution of toxic substances to quantitatively describe relationships among biological processes. They are used to estimate internal dose of a putative toxic moiety in a target tissue following various combinations of exposure route, dose level, and test species. PBPK models refine our understanding of complex dose relationships, such as external exposure concentration for a given species versus tissue dose of the toxicant and tissue dose versus observed adverse effect of the toxicant. In addition, PBPK models can be used to extrapolate pharmacokinetics from high to low doses, from one exposure route to another, from one species to another, and from one subpopulation to another within a species. PBPK models have been developed for a number of metals (e.g., manganese, arsenic, chromium, lead, mercury, and nickel) and are often used in risk assessment of these metals to predict tissue dose based on a given exposure and to extrapolate safe environmental exposure levels for humans. For example, PBPK models have recently been developed for manganese, first for rodents and nonhuman primates and more recently for humans, to determine target tissue dosimetry in various regions of the brain that are associated with neurotoxicity following inhalation exposure to high concentrations of manganese. Metal exposure and effect can be measured through the use of biomarkers. Biomarkers can be broadly categorized (as defined by the International Program of Chemical Safety) as follows: Examination of exposure to metals has typically been evaluated through analysis of urine, blood, hair, or other biological tissues. For example, blood lead monitoring was key to identifying and tracking exposure to lead in gasoline. As the use of lead decreased in the 1980s, a concomitant reduction in BLLs was observed in the U.S. population. Measurement of mercury concentrations in hair has routinely been used to document exposure to methyl mercury and generally corresponds to roughly 250 times the concentration measured in blood. Arsenic tends to accumulate in keratin-rich tissues, and exposure may manifest as discolored bands or Mees’ lines in fingernails and toenails. Finally, elevated exposure to silver compounds may result in a gray or blue–gray discoloration of the skin, termed argyria. Biomarkers of effect can be measured through biochemical, molecular, or behavioral indices. For example, impaired kidney function resulting from cadmium exposure may be identified by increased levels of serum proteins in the urine. Biomarkers for cancer development include tests for chromosomal alterations or changes in DNA, RNA, and protein expression. BLLs, in addition to being used as a determinant of exposure, are also used to monitor for cognitive impairment as BLLs at or below 10 μg/dl have been linked to deficits in IQ. Interpretation of effect biomarkers can be difficult because many biomarkers may not be specific to a single metal or chemical. Recent advances in toxicogenomics and molecular epidemiology are providing tools to identify genetic markers that signify susceptibility to a toxicant. For example, the protein metallothionein is important for the detoxification of metals. When normal levels of metallothionein are diminished due to metal exposure, adverse health effects may occur. Thus, measuring the gene expression of proteins such as metallothionein can provide a biomarker of susceptibility. Examination of genetic polymorphisms and other genetic biomarkers will enhance monitoring and diagnosis of environmentally related diseases. The interaction of metals with organs, tissues, cells, and cellular components can result in toxic effects. This may occur by a number of mechanisms that are specific to each metal. However, common toxic mechanisms of action for metals include the following categories: Essential nutrients are vitamins and trace elements that are critical for normal biological function (e.g., calcium, copper, sodium, and zinc). While nonessential metals are those elements that have no recognized beneficial biological role (e.g., cadmium, mercury, and lead). Some metals are essential for some animals and plants (e.g., boron, nickel, and silicon). Essential metals are components of proteins and enzymes that participate in homeostatic regulation of cellular function and metabolism, hormone levels, and growth and development (see Table 16.2). Essential metals have a “U”-shaped dose–response curve where low levels (i.e., nutrient deficiency) and high levels of metal exposure can disrupt the regulatory capacity of the organism. Recommended dietary reference intakes have been established for many essential metals (Table 16.2), and these represent a daily dietary intake sufficient to meet the nutrient requirements of nearly all healthy individuals. Tolerable upper intake levels (TULs) and chronic oral reference doses (RfDs) represent daily intakes that if exceeded can present a health risk from exposure to the metal (Table 16.2). Table 16.2 Health-Based Oral Intake Levels for Metals 1. “—” = none available. 2. Range for male and female adults. 3. Reference dose = An estimate (with uncertainty spanning perhaps an order of magnitude) of a daily oral exposure to the human population (including sensitive subgroups) that is likely to be without an appreciable risk of deleterious effects during a lifetime. It can be derived from an no observed adverse effect level (NOAEL), an LOAEL, or a benchmark dose, with uncertainty factors generally applied to reflect limitations of the data used. Generally used in US EPA’s noncancer health assessments. 4. Recommended daily intake = A daily dietary intake sufficient to meet the nutrient requirements of nearly all (97–98%) healthy individuals. 5. Tolerable upper intake level is the highest level of daily nutrient intake that is likely to pose no risk of adverse health effects to almost all individuals in the general populations. 6. Dose adjustments made based on a 70-kg adult. Essential metals can be toxic when intakes exceed required levels. This may occur after acute or chronic exposure that results in internal concentrations that exceed the body’s ability to regulate and excrete surplus metal ions. It is even possible that certain metal carcinogens, such as arsenic, may be essential. Interactions among essential and nonessential elements introduce complexity into the evaluation of metal toxicology. For example, selenium plays a role in alleviating toxicity due to mercury exposure and zinc can antagonize cadmium toxicity. Intake of zinc, sulfur, and iron are known to modulate copper deficiency or toxicity. Therefore, examination of the essentiality is critical for interpreting exposure and toxicity of metals. Arsenic naturally occurs in the environment in several different forms. Inorganic arsenic (i.e., as arsenite (As3+) or arsenate (As5+)) is the most common form of arsenic in soil, water, and air. On average, background concentrations of As in soil are approximately 10 mg/kg, while average concentrations in groundwater are less than 1 μg/l. Average concentrations in air away from a point source are generally under 5 ng/m3 in rural area and 20–30 ng/m3 in more urban settings (~20–30 ng/m3 overall). For the typical individual, diet is the largest source of inorganic arsenic exposure, with intake levels in the U.S. population ranging from about 1 to 20 μg/day. Grains, fruits, and vegetables typically contain the highest levels of inorganic arsenic. Seafood, the largest source of total arsenic in the diet, typically contains high levels of arsenobetaine, a nontoxic organic arsenic compound. Overall, human exposure to inorganic arsenic from these background sources is well below doses associated with adverse health effects. Several areas throughout the United States and the world, however, contain naturally occurring inorganic arsenic in drinking water, which constitutes a significant source of exposure (e.g., drinking water sources contain over several hundred micrograms per liter arsenic) and pose a public health concern. Organic arsenic compounds are also found in the environment. For example, arsenobetaine is a common constituent of seafood. The organic arsenic compounds monomethylarsonic acid (MMA) and dimethylarsinic acid (DMA) are also naturally occurring in environmental media, although usually at lower levels than inorganic arsenic. These two compounds are currently used as herbicides. Another form of arsenic with toxicological importance is arsine gas, which is formed when arsenic reacts with hydrogen gas. It is used in the semiconductor industry but also may be generated during the smelting and refining of metals, which involves treating the metals with acid. Arsine gas is a potent hemolytic agent (i.e., it breaks down blood cells). When inorganic arsenic is ingested or inhaled, it undergoes sequential reduction and methylation reactions and is ultimately extensively metabolized to MMA and DMA, which are excreted in urine with a whole-body half-life of few days. Arsenic excreted in urine is typically composed of 10–30% inorganic arsenic, 10–20% MMA, and 60–80% DMA, although there is significant interindividual variability likely due to polymorphisms is arsenic-metabolizing genes. In contrast, when organic arsenic compounds are ingested, they undergo limited cellular uptake and are excreted in urine largely without further metabolism. A key exception is in the rat, where ingestion of DMA results in relatively high amounts of a trimethylated arsenic metabolite (trimethylarsine oxide). While the methylation of arsenic has traditionally been considered a detoxification mechanism because it facilitates excretion, research over the past decade has shown that the process of arsenic metabolism produces unstable trivalent methylated intermediates. It is hypothesized that these intermediates play a role in the chronic adverse effects associated with arsenic exposure. The potent toxicity of arsenic has been well known for centuries; it has been used as a homicidal agent throughout history, purportedly in several high-profile political assassinations. Due to its toxic properties, it has also been used as a pest control agent. In particular, in the early 1900s, lead arsenate was used as a pesticide in apple orchards. Although the use of lead arsenic has been phased out, because of its environmental persistence, this legacy contamination still poses significant public health concerns in some areas of the United States. Some modern uses of arsenic-based pesticides still exist, including the selective use of copper chromated arsenate as wood treatment and the use of organic arsenicals as a herbicide for cotton. Most information regarding acute arsenic toxicity in humans derived from its medicinal use or from incidents in which individuals were poisoned with arsenic (either intentionally or accidentally). Signs of acute arsenic toxicity include encephalopathy, nausea, anorexia, vomiting, abdominal pain and diarrhea, dermatitis, muscle cramps, cardiovascular effects, hepatotoxicity, bone marrow suppression and hematologic abnormalities (anemia), vascular lesions, and peripheral neuropathy (motor dysfunction and paresthesia). Arsenic toxicity can often effectively be treated with the chelator 2,3-dimercapto-1-propanesulfonate or meso-2,3-dimercaptosuccinic acid. Information on the oral chronic effects of arsenic are mainly from observations where humans have been exposed to high concentrations of arsenic in drinking water. Heath effects from arsenic in soil have not been observed, likely because of the reduced bioavailability of arsenic in soils and because overall, exposures via soil pathways are relatively low. Based on several studies, an increase in arsenic body burden is not observed until arsenic concentrations in soil exceed several hundred micrograms per kilogram of soil.
PROPERTIES AND EFFECTS OF METALS
16.1 GENERAL CHARACTERISTICS OF METALS
16.2 ENVIRONMENTAL AND OCCUPATIONAL EXPOSURE
Natural State of Metals in the Environment
Soil
Domestic Groundwater
Food
Air
10th
90th
10th
90th
Typical Range
Typical Range
(mg/kg)
(µg/l)
(mg/day)
(ng/m3)
As
1.8
10
0.11
7.53
0.05
20
30
Be
0.5
2.1
<1
<1
No information
0.03
0.2
Cd
<0.1
0.5
<1
<1
0.03
1
40
Cr
10
52
0.17
4
0.06
10
30
Cu
5
23
0.17
12.3
0.9
1.3
5
200
Pb
9.6
27
0.01
1.09
Highly variable
Highly variable
Mn
163
1140
0.05
172.39
1
10
20
Mo
0.26
1.48
0.13
6
No information
No information
Hg
<0.02
0.08
nm
nm
0.0035
6
20
Ni
5.6
31
0.1
3
0.17
7
12
Se
<0.2
0.7
0.13
3.02
0.071
0.152
No information
Tl
0.2
0.7
<1
<1
No information
No information
Zn
22
90
1
99.86
7
16.3
100
1700
Transformations
Anthropogenic Exposures
Exposures from Naturally Occurring Metals
16.3 GENERAL PROPERTIES OF ABSORPTION, DISTRIBUTION, METABOLISM, AND EXCRETION OF METALS
Absorption and Distribution
Metabolism and Storage
Excretion
Chelation
Physiologically Based Pharmacokinetic Models
16.4 BIOMARKERS
16.5 GENERAL MECHANISMS OF TOXICITY
16.6 ESSENTIALITY VERSUS TOXIC EFFECTS
Recommended Dietary Allowance for Adults
Tolerable Upper Intake Level for Adults
Chronic Oral Reference Dose for Adults
Element
Essential role
(mg/day)
(mg/kg-day)
(mg/day)
(mg/kg-day)
(mg/day)
(mg/kg-day)
Arsenic
Not essential for humans
—
—
—
—
0.02
0.0003
Boron
Not essential for humans
—
—
20
0.3
14
0.2
Calcium
Essential for teeth and bone formation
1000
14
2500
36
—
—
Chloride
Principal anion needed to regulate extracellular fluid volume and plasma osmolality
2300
33
3600
51
—
—
Chromium
Glucose and insulin metabolism
0.025–0.035
0.0004–0.0005
—
—
150 (Cr3+)
1.5 (Cr3+)
0.2 (Cr6+)
0.003 (Cr6+)
Cobalt
Component of vitamin B12, necessary for maintenance of hematological status
—
—
—
—
—
—
Copper
Component of metalloenzymes
0.9
0.01
10
0.14
—
—
Fluoride
Associated with calcified tissues and prevents dental caries
3–4
0.04–0.06
10
0.14
4.2
0.06
Iodine
Component of thyroid enzymes
0.15
0.002
1.1
0.02
—
—
Iron
Component of proteins (e.g., hemoglobin, myoglobin, and ferritin)
8–18
0.1–0.3
45
0.6
—
—
Magnesium
Cofactor for enzymes involved in glycolysis and oxidative phosphorylation
320–420
4.6–6.0
350
5
—
—
Manganese
Component of metalloenzymes important for bone formation and cholesterol, and carbohydrate metabolism
1.8–2.3
0.026–0.033
11
0.16
9.8
0.14
Molybdenum
Cofactor for enzymes involved in catabolism of sulfur amino acids
0.05
0.0006
2
0.03
0.35
0.005
Nickel
Not essential for humans
—
—
1
0.01
1.4
0.02
Phosphorous
Component of biological membranes and supports tissue growth
700
10
4
0.06
—
—
Potassium
Major intracellular cation necessary for normal function
4700
67
—
—
—
—
Selenium
Component of proteins important for defense against oxidative stress and regulation of other vitamins and hormones
0.06
0.0008
0.4
0.006
0.35
0.005
Sodium
Principal cation needed to regulate extracellular fluid volume and plasma osmolality
1500
21
2300
33
—
—
Vanadium
Not essential for humans
—
—
1.8
0.03
—
—
Zinc
Component of or catalyst for a number of enzymes
8–11
0.11–0.16
40
0.57
21
0.3
16.7 TOXICOLOGY OF SPECIFIC METALS
Arsenic
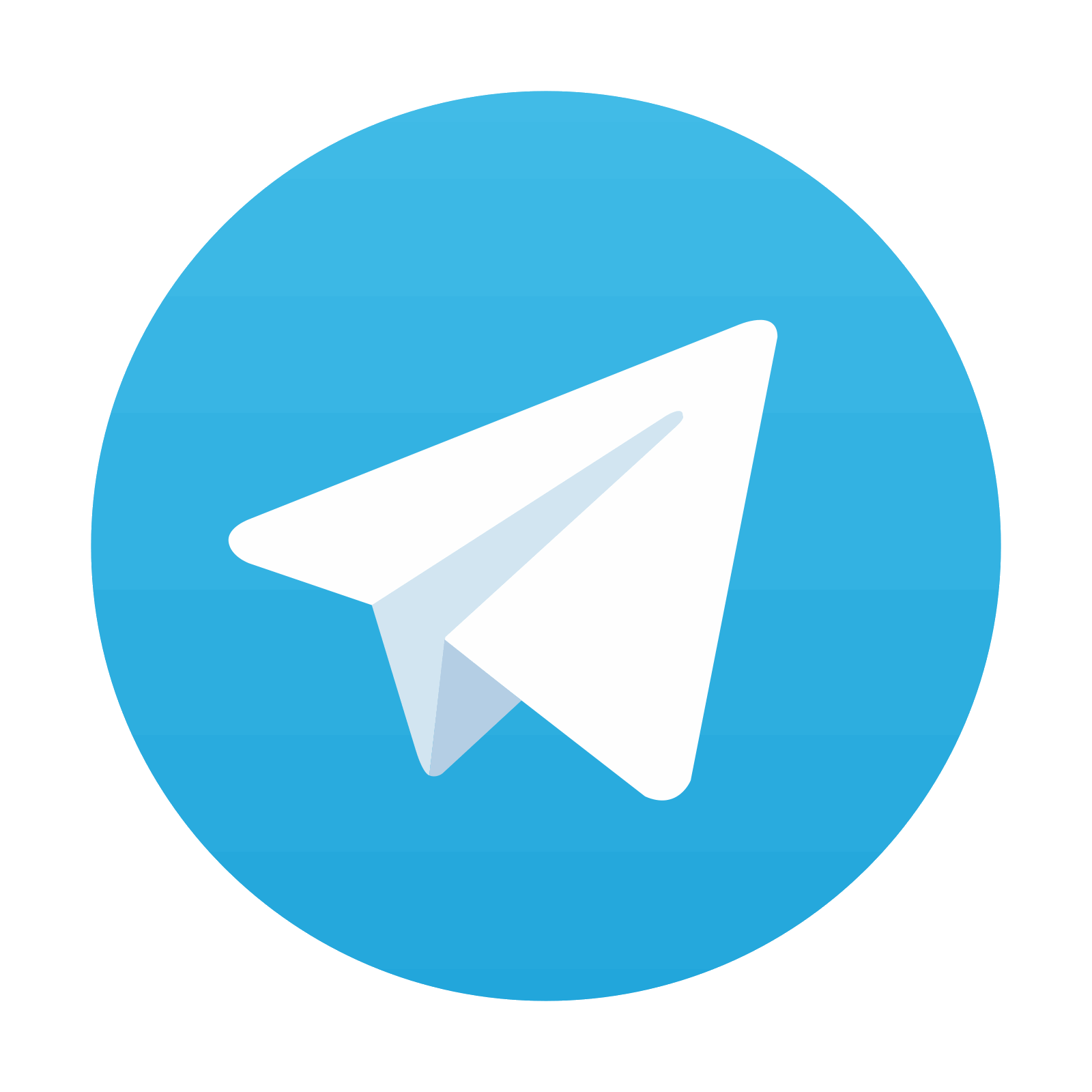
Stay updated, free articles. Join our Telegram channel
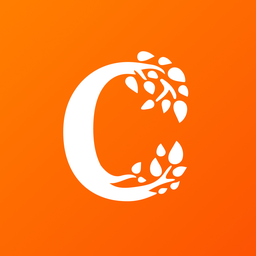
Full access? Get Clinical Tree
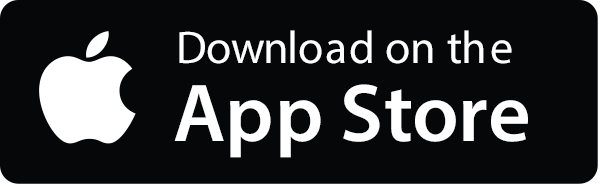
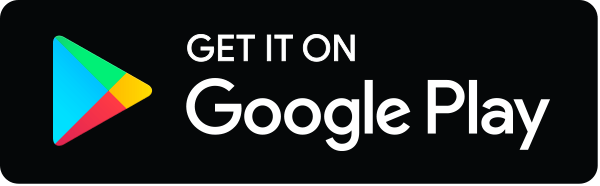