Fig. 20.1
Schematic diagram of immune cells involved in post-stroke inflammation in the brain. Immune cells infiltrate the ischemic hemisphere and are thought to promote cell injury and death via the release of various cytokines and effector molecules. Upward arrow increased; downward arrow decreased
Neutrophils are one of the most abundant immune cells to infiltrate the brain following stroke. Once in the brain, neutrophils undergo granule exocytosis to release a variety of pro-inflammatory molecules, including large quantities of nitric oxide (NO) derived from inducible NO synthase, nicotinamide adenine dinucleotide phosphate (NADPH) oxidase-derived ROS, and matrix metalloproteinases, which subsequently contribute to neuronal damage and BBB permeability [10]. Interestingly, recent evidence suggests that pro-inflammatory cytokines derived from infiltrating T lymphocytes may attract neutrophils to the ischemic area following stroke [13, 14].
Both CD4+ and CD8+ T lymphocytes are major contributors to brain inflammation following cerebral ischemia. CD4+ T cells can be further subdivided into type 1 helper cells (Th1), Th2 cells, Th17 cells and T regulatory cells, based on their functional and cytokine profiles [8, 15]. Th1 cells are pro-inflammatory and release a variety of cytokines such as interferon-γ (IFN-γ), interleukin-1 β (IL-1 β), and tumor necrosis factor (TNF), which can promote inflammation and cell death [15]. By contrast, Th2 cells are anti-inflammatory and release cytokines such as IL-4, IL-5 and IL-10, which can suppress inflammation and the immune response following stroke [8]. Importantly, mice deficient in Th1 cells have reduced stroke-induced infarct damage whereas mice deficient in Th2 cells have exacerbated injury after cerebral ischemia [14]. Furthermore, cytokines released from Th1 and Th2 cells are capable of driving macrophage polarization to either an activated “M1” or an alternately activated “M2” state, respectively [16]. It is important to note that macrophages and/or microglia that assume an M1 phenotype produce a host of pro-inflammatory cytokines, whereas M2 macrophages and/or microglia probably play a more protective role via the release of anti-inflammatory mediators. It is therefore conceivable that future therapies that alter the balance away from the Th1/M1 and toward the Th2/M2 immune profile may be beneficial for stroke.
The role that CD4+ T regulatory cells (Tregs) play in stroke outcome has received growing interest. Whilst there is some evidence to suggest that Tregs may be cerebroprotective following stroke [17], others indicate they play a minimal [14, 18] or even a detrimental role [19]. Discrepancies between such studies may be explained by specific differences in experimental models or acute versus chronic differences in the role of Tregs after stroke. Further investigations are needed to more fully elucidate the role of Tregs following stroke, particularly to examine both acute and chronic time points after cerebral ischemia.
It is important to highlight that CD4+ T cells are not the only T cell subset thought to influence stroke outcome. CD8+ cytotoxic T lymphocytes are reported to contribute to infarct formation following stroke [14, 20] and, moreover, have the ability to induce apoptosis in already compromised neuronal cells via release of cytotoxins and activation of the Fas receptor [15, 21]. By contrast, the influence that B lymphocytes have on stroke outcome remains controversial. A number of studies have found no evidence that B cells affect infarct formation post-ischemia [20, 22], despite B cells being part of the cellular infiltrate into the brain following stroke [23]. On the other hand, Ren et al. [24] reported that stroke injury is augmented in B-cell-deficient mice compared to wild-type animals. The protective effect mediated by B cells appears to be dependent upon IL-10 secretion, as adoptive transfer of B cells from IL-10-deficient mice had no influence on stroke outcomes [24] while transfer of IL-10 producing B cells to B-cell-deficient mice was found to be protective [25].
4 The Systemic Immune Response Following Stroke
The immune response following stroke is not limited to the brain, with profound effects on immune function also seen in the periphery. These effects include an early activation phase that lasts for 24–48 h, followed by a severe systemic immunosuppression. It is during the early phase that the spleen plays a primary role in the influx of immune cells. For example, Ajmo and colleagues showed that splenectomy prior to experimental stroke reduces infarct size [26]. Moreover, splenectomy-induced neuroprotection following stroke correlated with a decrease in activated microglia, macrophages and neutrophils in the ischemic hemisphere, indicating that the spleen may be a source of detrimental immune cells following stroke [26]. In addition, Offner et al. reported that T lymphocytes, derived from blood and natural killer (NK) lymph nodes, secreted increased levels of pro-inflammatory mediators and expressed elevated levels of chemokine receptors following stroke [27]. More recently, it was shown that the spleen contributes to neurodegeneration through IFN-γ signaling following stroke [28].
Following the initial response, a profound systemic immunodepression or “stroke-induced immunodeficiency syndrome” occurs [15, 29, 30]. This phenomenon is generated by hyperactivity of the sympathetic nervous system and the hypothalamic–pituitary–adrenal axis due to post-stroke brain damage, which leads to decreased numbers of T and B lymphocytes and also of natural killer (NK) cells within the spleen, thymus, bone marrow and lymph nodes [17, 27, 29, 30]. This results in increased apoptosis and increased release of immune cells from these primary and secondary lymphoid organs, resulting in tissue atrophy, which predisposes patients to infection (e.g., commonly resulting in pneumonia or sepsis), a major determinant of stroke morbidity and mortality.
Although the liver is not a secondary lymphoid organ, an important recent study investigated the effect stroke had on invariant natural killer T (iNKT) cells within the liver [31]. Wong and co-authors showed that cerebral ischemia–reperfusion slowed the migration of resident hepatic iNKT cells in the liver and increased the expression of the immunosuppressive cytokine, IL-10, in association with an increased susceptibility to bacterial infection. In support of these findings, the onset of bacterial infection occurred much earlier in iNKT cell-deficient mice following stroke, whereas cerebral infarct damage was unchanged. This indicated that iNKT cells play an important role in systemic protection and ultimately survival following infection after stroke. Furthermore, the authors found that the increased release of noradrenergic neurotransmitters from sympathetic nerves innervating the liver following stroke can undermine systemic immunity by a direct inhibitory effect on hepatic iNKT cells. Hence, the authors suggested that blockade of stress pathways could improve outcomes in stroke patients by helping to protect systemic immune function and thus preventing infections. Collectively, evidence indicates that various components of the immune system in the brain as well as in secondary lymphoid and visceral organs may play vital roles in the development of post-stroke outcomes.
5 The Potential for Stem Cell Therapy in Stroke
Given the complex, time-dependent molecular mechanisms of injury following cerebral ischemia, as well as the numerous cell types apparently involved, ultimately successful approaches could include cell-based therapies that have the potential to target various injury mechanisms and cell types when administered at an appropriate time(s) after the stroke event. Hence, there is now considerable interest in stem cell therapy as a potential treatment for stroke. Stem cells are undifferentiated cells capable of self-renewal and are broadly classified as being of embryonic, fetal or adult origin [32]. Embryonic stem cells (ESCs) are pluripotent, meaning they can give rise to all cell types of an organism, whereas fetal and adult stem cells are multipotent, such that they can give rise to cells of multiple, but limited numbers of lineages. A number of stem cells, including embryonic, bone marrow, neural and induced pluripotent stem cells (iPSCs) have been shown to improve experimental stroke outcome [33–36]. As a result of such promising preclinical findings, the first fully regulated clinical trial (PISCES study—Pilot Investigation of Stem Cells in Stroke) using a manufactured neural stem cell line to treat stroke patients is underway in Glasgow. Unfortunately, however, there tends to be major limitations with the use of most stem cell types, which may offset their use as a feasible routine treatment for stroke patients.
6 Limitations and Benefits of Stem Cell Lineages for Transplantation
Initially, ESCs were expected to have broad therapeutic potential due to their pluripotent capabilities, and transplantation of human ESC neural derivatives into a rodent model of stroke has been reported to improve functional outcome [33, 35]. Nevertheless, multiple problems exist regarding human ESCs, including ethical/political issues (i.e., due to the destruction of human embryos), immune rejection, and their fetal “age” (i.e., they lack key functional characteristics of adult cells) [37]. Moreover, ESCs may form teratomas (developmental tumors) following transplantation [38].
iPSCs were first derived in 2006 [39] by re-programming mouse and human fibroblasts into pluripotent ESC-like cells. Since then, many types of iPSCs have been created using diverse cell types [40]. iPSCs possess most of the important properties of ESCs but avoid the ethically controversial issues surrounding embryo destruction. These cells have been used to treat central nervous system (CNS) injuries such as spinal cord injury and stroke in rodents [36, 41], but in both cases tumor formation from iPSCs was discovered.
Fetal neural stem cells (NSCs) are isolated from human fetal brains (derived from aborted material) and are capable of differentiating into neurons, astrocytes, or oligodendrocytes [42]. Due to the invasive nature of extracting autologous adult human neural cells, fetal NSCs are considered as an alternative expandable source of neural cells. Although NSCs also involve ethical issues, these cells were thought to be safer than human ESCs regarding tumor formation after transplantation, however brain and spinal cord tumors have been shown to develop following fetal NSC treatment [43–45].
Mesenchymal stem cells (MSCs), derived from bone marrow or umbilical cord blood, can differentiate into neuronal-like cells, astrocytes or endothelial cells, and their administration can decrease infarct size and improve functional outcome in preclinical models of stroke [46, 47]. Transplantation of MSCs can also reduce apoptosis and promote endogenous cellular proliferation after stroke, and long-term follow-up data have revealed improved survival in patients that received bone marrow MSCs compared with controls [48]. Similar to ESCs, however, concerns and limitations associated with MSC use in stroke include poor cell survival and engraftment after transplantation, no direct evidence of functional neuronal differentiation, limited sources, and the fact that their removal from bone marrow requires invasive procedures, although they do not appear to form tumors after transplantation [49].
7 Human Amnion Epithelial Cells
7.1 hAEC Characteristics
While the above stem cell lineages may have therapeutic potential if their respective issues can be addressed, currently those limitations seriously offset their likely routine use in clinical stroke. An alternative stem cell type that is gaining recognition as a potential therapeutic are human amnion epithelial cells (hAECs). hAECs are derived from the amniotic sac, a thin avascular tissue that encloses the fetus and is attached to the placenta. The innermost layer of the amnion comprises epithelial cells that are in direct contact with the amniotic fluid. It is this amniotic epithelial layer that plays a critical role in providing the embryo with an optimal amniotic environment in which the embryo can develop.
It has been known for more than 30 years that hAECs are safe to transplant into humans [50] and they have a number of properties that make them ideal as a potential therapeutic. For example, they are anti-inflammatory [51], able to modulate host immune cell responses to injury [52], and are anti-fibrotic [53]. Indeed, amnion cells or strips of amnion membrane have long been used to reduce scarring and promote healing in burn victims [54] or following corneal surgery [55]. It is thought that the ability of hAECs to promote “scar-free” healing underlies the ability of the fetus to heal without scarring [56, 57]. hAECs are also pluripotent, including being able to differentiate down neural lineages [58]. More specifically, hAECs may express markers of glial and neuronal progenitor cells, and display multiple neuronal functions, such as synthesis and release of acetylcholine, catecholamines, and neurotrophic factors [59, 60]. Important for any future clinical therapy, and unlike ESCs, hAECs do not form tumors in vivo [50, 61], nor do they differentiate into fibroblasts as MSCs can [62], suggesting that hAECs are an inherently safer cell therapy than these other cells. Further, likely reflecting their role in maintaining maternal immune tolerance of the allograft that is the fetus in pregnancy, hAECs are immunologically inert. Primary hAECs express and release the non-polymorphic, non-classical human leukocyte antigen G (HLA-G) and lack surface expression of the polymorphic antigens HLA-A, HLA-B and HLA-C (class IA) and HLA-DR (class II) [50]. Indeed, when hAECs were administered to healthy volunteers there was no evidence of immune rejection [50]. This is in keeping with the burns and ophthalmology literature where extensive allogeneic use of amnion has occurred without rejection. Moreover, hAECs do not express telomerase and have telomere lengths more in keeping with ESCs than adult bone marrow MSCs [63]. Lastly, with approximately four million births a year (US statistics) and an average yield of 150–200 million cells per placenta [63], hAECs are abundant and relatively easy and cheap to harvest. As such, hAECs are readily available, they require no invasive procedure for harvesting, and they lack major ethical barriers to their use [32].
7.2 hAECs in the Treatment of Stroke
From the information above, it is not surprising that interest in hAECs as a source of allogeneic cells for regenerative therapies is growing [64]. In animal models of CNS disorders, accumulating evidence suggests that hAECs can exert neuroprotection and facilitate neuroregeneration [65–73]. Conversely, only one published study has tested the effect of hAECs on ischemic stroke outcome [74]. It found that direct intra-cerebral (i.c.) injection of hAECs, 24 h after middle cerebral artery occlusion (MCAO) in rats, resulted in a reduced infarct volume and improved behavioral and neurological outcomes at 16 days post-stroke. Furthermore, expression of the key marker of apoptosis, cleaved caspase-3, was reduced in the vicinity of the transplanted cells. The same research group has also reported that i.c. injection of human amnion mesenchymal cells can similarly improve stroke outcome in rats [75]. In analogous studies to those in ischemic stroke, intraventricular injection of hAECs has been reported to reduce brain edema and to improve motor deficit in a rat model of intra-cerebral hemorrhage [76]. Moreover, intra-cerebroventricular transplantation of amniotic fluid-derived stem cells at 3 days post-MCAO resulted in the attenuation of stroke-associated cognitive deficits [77]. Despite these very promising early experimental findings, i.c. injection of stem cells is not attractive as a clinical therapy for stroke patients. This is because i.c. injections would require ready access to suitable imaging facilities and surgical expertise, and could even involve significant adverse effects, such as the breakdown of the BBB and a heightened inflammatory response within the brain [78]. Furthermore, any protective effects from i.c. injection of stem cells would presumably be localized to the immediate region of brain and would not target any of the additionally detrimental stroke-induced effects occurring in the periphery. Therefore, future studies would ideally employ a less invasive and more clinically amenable delivery route of stem cells, such as intravenous (i.v.) injection.
As i.v. injection is a minimally invasive procedure, it poses a substantially lower risk of adverse clinical events when compared to i.c. transplantation and, due to the acute nature of stroke onset, an i.v. injection is ideal so that therapeutics can be administered quickly after the event. However, i.v. administration of stem cells has two initial obstacles that must be overcome: (1) the ability of the cell to pass through the extensive capillary network of the lungs; and (2) whether the cells can effectively home to stroke-affected regions of tissue in sufficient numbers to provide efficacy. Whether this may occur remains to be tested, but the relatively small diameter of hAECs approximately 15 μm) probably increases the likelihood of these cells passing through the lungs, compared with larger stem cell lineages, such as MSCs, which do not easily passage across the lungs [79]. Indeed, we have reported that only a minor percentage of i.v.-injected hAECs persist in the lungs, even if lung injury has been induced using bleomycin in mice [53]. Thus, it is conceivable that i.v.-administered hAECs may have minimal impact on lung function and that a substantial proportion of these cells can pass into the systemic circulation. In fact, Tarjiri and colleagues have reported that i.v. administration of amniotic fluid-derived stem cells at 35 days post-stroke significantly reduces infarct damage and behavioral deficits assessed at 60–63 days [80]. Hence there is considerable scope to further explore the ability of hAECs to limit injury and/or promote tissue repair and functional recovery when administered systemically following stroke.
7.3 Potential Mechanisms of Action by hAECs in the Treatment of Stroke
There are numerous possible mechanisms by which hAECs could exert therapeutic effects following stroke. Firstly, hAECs could secrete neurotrophic factors that promote recovery of damaged neurons [74, 81]. Such factors could also stimulate synaptogenesis to re-innervate lost connections. Secondly, as hAECs have pluripotent properties, they could differentiate into a neuronal phenotype and replace injured or dead cells [74, 81]. Thirdly, hAECs could act as a “mobile pharmacy,” secreting necessary cytokines, growth factors, hormones and/or neurotransmitters to restore cellular function. Lastly, hAECs could potentially improve stroke outcome by modulating the immune response that contributes to brain injury [81, 82]. Included in this mechanism is the protection of neurons from immune cell-mediated apoptosis.
7.4 Immunomodulatory Properties of hAECs
While it was initially proposed that stem cells exerted their reparative effects by integration and differentiation in vivo, growing evidence suggests that it is more likely that they work by modulating the host immune response to cease inflammation and coordinate resolution and repair (see Fig. 20.2). hAECs can exert immunomodulatory actions by actively suppressing T and B lymphocyte proliferation [83] reducing the expression of the potent pro-inflammatory cytokines IL-1α and IL-1β [84], and via producing inhibitors of MMPs and proteolytic enzymes associated with inflammatory reactions. In addition, although expression of HLA-G on hAECs enables their evasion of the immune system, this protein has also been shown to be anti-inflammatory by inducing apoptosis of activated CD8+ T lymphocytes and inhibiting CD4+ T lymphocyte proliferation [85]. Furthermore, hAECs transplanted to the ocular surface can create a local environment that reduces the surrounding inflammatory response [86]. This effect is thought to be due to hAECs reducing infiltration of major histocompatibility complex class II antigen-presenting cells into the inflamed cornea. Furthermore, our group has demonstrated that hAECs transplanted into a bleomycin-induced lung injury model reduces the immune response, preventing lung fibrosis and loss of function [53, 87]. These results were associated with an in vivo reduction in the pro-inflammatory cytokines, TNF, IFNγ and IL-6, and an increase in the anti-inflammatory cytokine, IL-10 [87]. As a consequence of these actions of hAECs on the immune system, there was a reduction in the infiltration of immune cells to the area of damage. In a follow-up study, we found that hAECs reduce macrophage infiltration into the bleomycin-injured lungs, reducing M1 (CD86+) macrophages but increasing M2 (CD206+) macrophages [52]. This is likely to be important to injury repair processes because, as described earlier, M1 macrophages are pro-inflammatory and are involved in the acute inflammatory response, whereas M2 macrophages are immunosuppressive and pro-reparative, coordinating the termination of inflammation, debris scavenging and angiogenesis [88]. Consistent with this, we have also shown that hAECs reduce macrophage migration and stimulate macrophage phagocytosis in vitro—features of an M2 phenotype [52].


Fig. 20.2
Schematic diagram depicting the various immunomodulatory actions of human amnion epithelial cells (hAECs). It is via these mechanisms that hAECs may improve stroke outcome. Upward arrow increased, downward arrow decreased, neuts neutrophils, macs macrophages
With regard to the interactions with T cells, we postulate that hAECs could drive naïve T cells into FoxP3+ Tregs, consistent with a reparative response to injury, analogous to what occurs in pregnancy where the placental/fetal cells manipulate maternal immune function to prevent fetal rejection by biasing maternal immunity away from Th1 and toward Th2 activity—the so-called Th1–Th2 tilt [89]—and where the resultant Tregs drive macrophage polarity to M2 [89]. Thus, if hAECs ultimately influence macrophages to take on an M2 phenotype, and T cells to a Treg phenotype, such actions would be expected to be central to their reparative and protective effects, irrespective of where the injury is. Whether hAECs can provide neuroprotection via similar mechanisms following stroke remains to be established. Tregs certainly enter the brain following stroke where they appear to modulate tissue injury [15]. This is likely to involve the direction of non-Treg CD4+ T cells (T helper cells) toward a Th2-type anti-inflammatory response and away from a Th1 pro-inflammatory response [15]. Whilst there is good evidence for significant macrophage infiltration into the post-stroke brain [90], the extent of damage caused by macrophages, the identity of their phenotype/s (i.e., M1 or M2), or whether their phenotype can be manipulated to alter injury repair remains to be determined.
hAECs are thought to secrete a number of immunomodulatory factors. In fact, supernatant from hAEC culture can inhibit both innate and adaptive immune cells [83]. For example, hAECs produce alpha-fetoprotein, a protein that reduces immune cell reactivity and suppresses neuroinflammation in a mouse model of multiple sclerosis [91]. Furthermore, hAECs secrete macrophage inhibitory factor, which inhibits neutrophil and macrophage migration and natural killer cell-mediated cytolysis [83]. Fas ligand and TNF-related apoptosis-inducing ligand are both members of the TNF family that are produced by hAECs, and can regulate the immune response through apoptosis of lymphocytes [83]. Moreover, hAECs express transforming growth factor-β, which suppresses immune cell numbers through apoptosis [83]. Overall, the immunomodulatory properties of hAECs lead us to speculate that these stem cells may be able to limit the inflammatory response that contributes to infarct formation following stroke.
7.5 Migration of Intravenously Injected hAECs After Stroke
Stem cells communicate with each other and their environment via paracrine signaling [92]. In order to understand why and how cells migrate to their target organs, the relevant chemotactic signal(s) must be identified. Very little is so far known about the chemotaxis response involved in hAEC migration following administration. However, several studies have identified the mechanisms that attract other lineages of stem cells to injured sites following stroke. For example, there is an increase in stromal cell-derived factor-1α (SDF-1α) in brains of experimental animal models of stroke [93, 94] and a subsequent decrease in stem cell migration after the addition of an antagonist of the chemokine receptor type 4 (CXCR4) [94, 95]. SDF-1α is a growth factor produced by multiple types of mouse and human neural cells, and which functions as a chemokine that is thought to be important for neural progenitor migration during development. It is well established that the chemokine interaction between SDF-1α and CXCR4, its cognate receptor that is commonly expressed on the surface of stem cells, plays a major role in stem cell migration [94, 95]. Further research is required to clarify whether CXCR4 and/or other factors play a role in hAEC homing and signaling pathways.
7.6 Ability of hAECs to Engraft and Differentiate and/or Promote Neuronal Repair
Stem cell therapy was originally thought to be an opportunity to treat stroke patients in a manner that ultimately replaced dead neurons with new neurons in the infarcted region of brain. As indicated, hAECs can indeed differentiate toward a neural lineage, which may add to their potential for post-stroke therapy [32, 59, 60]. In fact, i.c. injections of hAECs in rats at 24 h after MCAO were found to migrate to ischemic areas and to then express astrocyte (glial fibrillary acidic protein) and neuronal markers (microtubule-associated protein 2 and nestin) [74]. Correlating with these observations, hAEC-treated rats showed improved behavioral and neurological outcomes, as well as reduced infarct. Thus, the authors suggested that the functional improvement following hAEC treatment may have been partially due to the newly differentiated neuron-like cells re-establishing connections with surviving host neurons. Similarly, in a hemorrhagic stroke model, hAECs transplanted into the brain were found to express neuron-specific markers and to improve motor deficits after 4 weeks [76]. As a further example, i.v. administration of amniotic fluid-derived stem cells resulted in an increased number of cells expressing microtubule-associated protein 2, and the cell proliferation marker, Ki67, in the dentate gyrus and in the subventricular zone of animals subjected to stroke, indicating increased neurogenesis [80]. Collectively, the existing evidence supports the concept that hAECs can undergo neural differentiation in vivo. Future studies must determine if these newly formed neurons are functional and able to integrate within the existing network of cells to substantially replace dead tissue.
As indicated above, secreted paracrine factors may also play a critical role in hAEC-mediated recovery after stroke. If administered as a delayed post-stroke therapy, hAECs could improve long-term stroke outcome via the release of factors that promote re-innervation, thus restoring synaptic transmitter release to stimulate plastic responses, orchestrating rescue and repair processes, and improving or preserving survival and function of existing neurons. Indeed, it has been shown that hAECs can release trophic factors such as brain-derived neurotrophic factor, neurotrophin-3, nerve growth factor [96, 97] and novel epidermal growth-like factors [98]. Whilst repair of damaged neurons following stroke has yet to be described, hAECs have shown an ability to promote recovery of injured tissue and facilitate functional plasticity in other CNS diseases. For example, transplanted hAECs produce neurotrophic substances and stimulate repair and regeneration of host neurons in a primate model of spinal cord injury [69]. In addition, hAECs transplanted into the cerebral lateral ventricle of a transgenic mouse model of Alzheimer’s disease were found to rescue damaged cholinergic neurons [99]. The authors reported that hAEC treatment increased the number of cholinergic neurons, as well as the level of acetylcholine produced by these cells, which was suggested to be largely responsible for the reversal of cognitive decline in this animal model. Therefore, hAECs may possess the ability to both repair and replace lost neuronal tissue and, together with their other anti-inflammatory characteristics, they may represent a very promising cell-based clinical therapy for neurodegenerative diseases, including stroke.
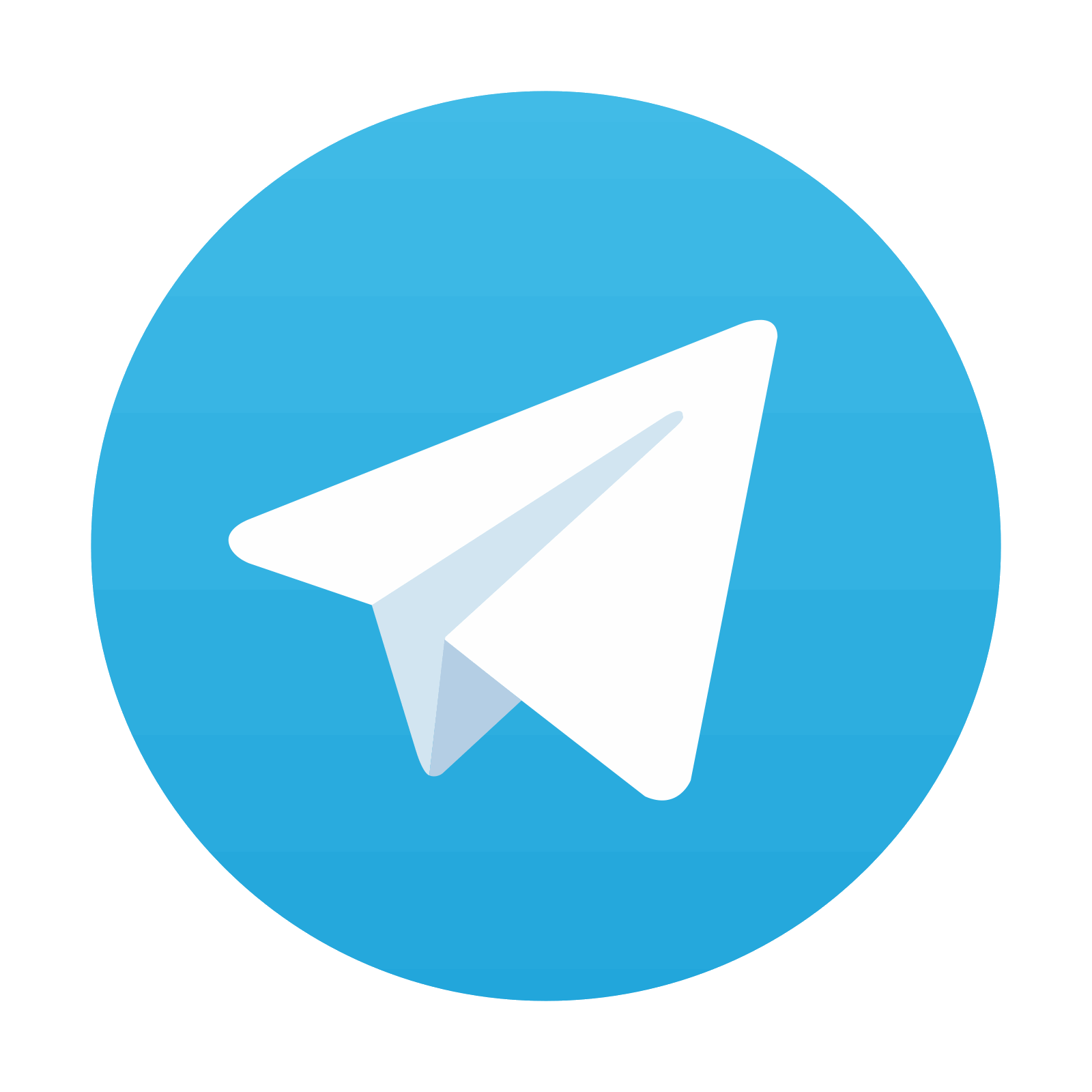
Stay updated, free articles. Join our Telegram channel
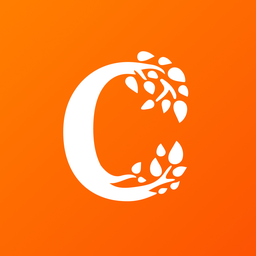
Full access? Get Clinical Tree
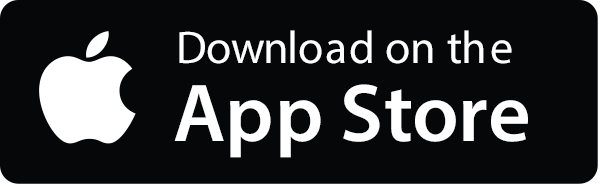
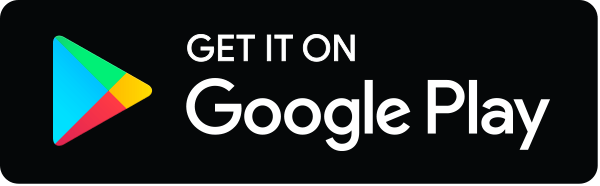