Physiology of the Respiratory System
RESPIRATORY PHYSIOLOGY
Functionally, the respiratory system is composed of an integrated set of regulated processes that include the following:
Transport of gases by the blood
Internal respiration: gas exchange in the systemic blood capillaries and cellular respiration
Figure 27-1 summarizes the essential processes of pulmonary function. We will use this set of processes as a general framework for this chapter. Cellular respiration has already been covered in Chapter 4 and will be reviewed again in greater detail in Chapter 30.
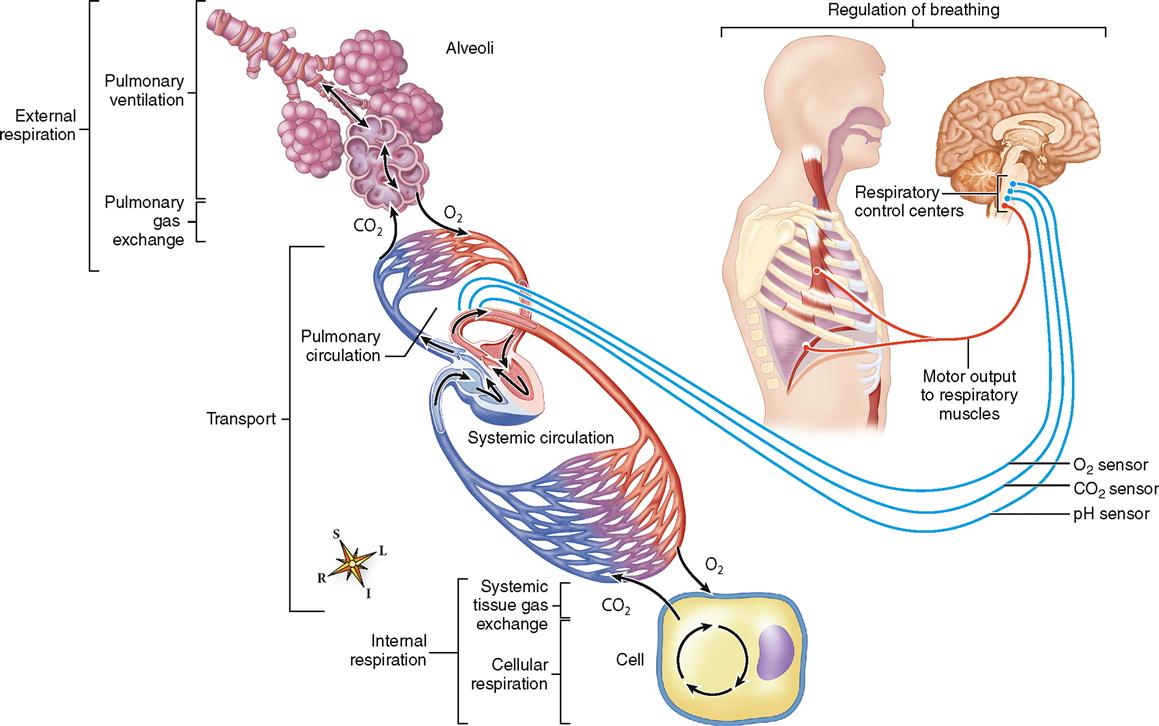
PULMONARY VENTILATION
Pulmonary ventilation is a technical term for what most of us call breathing. One phase of it, inspiration, moves air into the lungs and the other phase, expiration, moves air out of the lungs.
Mechanism of Pulmonary Ventilation
Air moves in and out of the lungs for the same basic reason that any fluid (a liquid or a gas) moves from one place to another—briefly, because its pressure in one place is different from that in the other place. Or stated differently, the existence of a pressure gradient (a pressure difference) causes fluids to move. A fluid always moves down its pressure gradient. This means that a fluid moves from the area where its pressure is higher to the area where its pressure is lower. When applied to the flow of air in the pulmonary airways, we can call this central idea the primary principle of ventilation.
Under standard conditions, air in the atmosphere exerts a pressure of 760 mmHg. Air in the alveoli at the end of one expiration and before the beginning of another inspiration also exerts a pressure of 760 mmHg. This explains why, at that moment, air is neither entering nor leaving the lungs. The mechanism that produces pulmonary ventilation is one that establishes a gas pressure gradient between the atmosphere and the alveolar air.
When atmospheric pressure is greater than pressure within the lung, air flows down this gas pressure gradient. Then air moves from the atmosphere into the lungs. In other words, inspiration occurs. When pressure in the lungs becomes greater than atmospheric pressure, air again moves down a gas pressure gradient. But this time, the air moves in the opposite direction. That is, air moves out of the lungs into the atmosphere. The pulmonary ventilation mechanism, therefore, must somehow establish these two gas pressure gradients—one in which alveolar pressure (PA, pressure within the alveoli of the lungs) is lower than atmospheric pressure (or barometric pressure, PB) to produce inspiration and one in which it is higher than atmospheric pressure to produce expiration. See Figures 27-2 and 27-3.
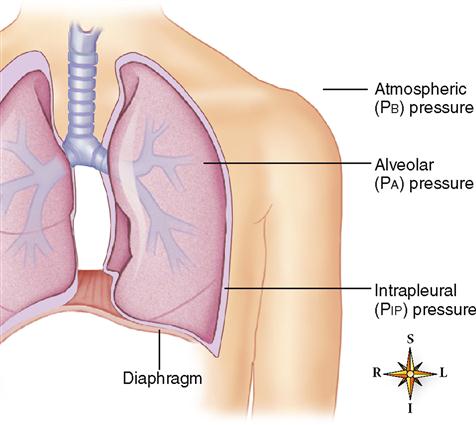
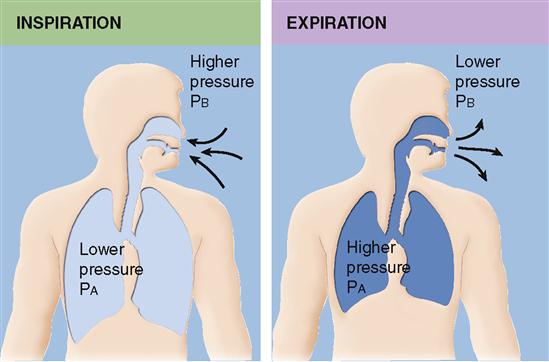
These pressure gradients are established by changes in the size of the thoracic cavity, which in turn are produced by contraction and relaxation of respiratory muscles. An understanding of Boyle’s law is important for understanding the pressure changes that occur in the lungs and thorax during the breathing cycle. It is a familiar principle, stating that the volume of a gas varies inversely with pressure at a constant temperature (Box 27-1). One application of this principle is as follows: expansion of the thorax (increase in volume) results in a decreased intrapleural (intrathoracic) pressure. This leads to a decreased intraalveolar pressure that causes air to move from the outside into the lungs.
The mechanics of ventilation are often modeled using a balloon in a jar, as you can see in Figure 27-4. The bell-shaped jar represents the rib cage (thoracic cavity), and a rubber sheet across the open bottom of the bell jar represents the diaphragm. A balloon represents the lungs. The space between the balloon and the jar represents the intrapleural space. Expanding the thorax by pulling the diaphragm downward increases thoracic volume—thus decreasing intrapleural pressure (PIP). Because the balloon is compliant (stretchable), the decrease in PIP causes a similar decrease in the balloon pressure (alveolar pressure, PA). This creates a pressure gradient that results in flow of air into the balloon. The opposite occurs when the elastic diaphragm recoils, decreasing internal air volumes (thus increasing internal air pressure) and forcing air out of the balloon.
Figure 27-5 applies the same principles of the balloon model to the human airways to demonstrate the mechanics of ventilation. The constant alternation between inspiration and expiration is called the respiratory cycle. The specific mechanics of the respiratory cycle are outlined in the following sections and in Table 27-1.
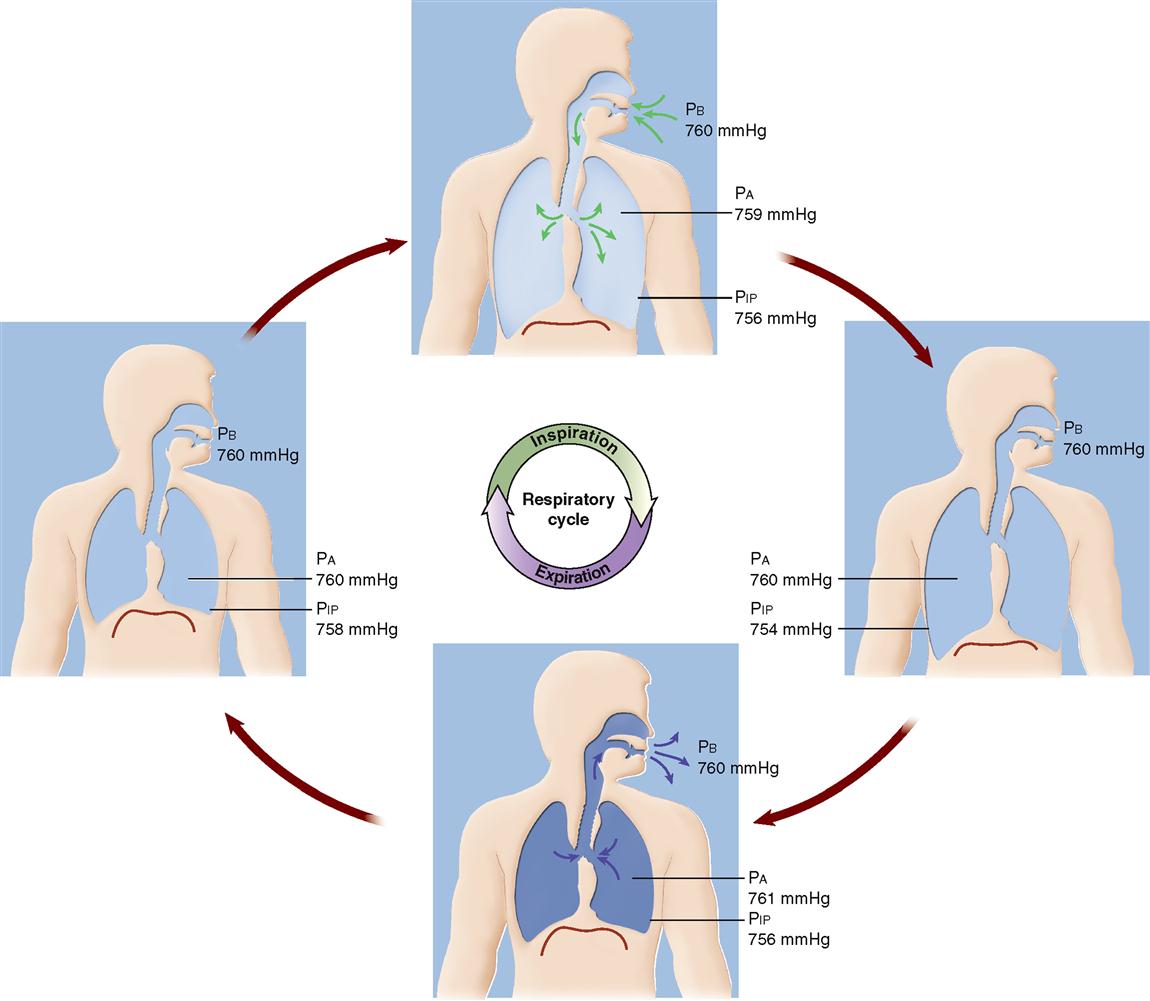
TABLE 27-1
PIP | PA | PB | DESCRIPTION |
Inspiration | |||
758 | 760 | 760 | The diaphragm is relaxed, putting the thoracic cavity at low volume. At the beginning of inspiration PIP < PA, keeping alveoli open. Since PA = PB, no air is flowing yet. |
756 | 759 | 760 | The diaphragm contracts, increasing the thoracic volume and reducing PIP. A decrease in PIP causes a decrease in PA. Now PA < PB, and air flows down the pressure gradient (into the lungs). |
754 | 760 | 760 | Eventually, the alveoli fill with air and PA equilibrates with PB. Inward airflow stops. The cycle is now ready to shift to the expiration phase. Note that PIP is still dropping but PA has not yet “caught up” with the drop. |
Expiration | |||
754 | 760 | 760 | As expiration is about to begin, the diaphragm is contracted maximally. Since PA = PB, there is no airflow. |
756 | 761 | 760 | The diaphragm relaxes, and elastic recoil of the thoracic walls and alveoli increases PIP and PA. Now, PA > PB. Air moves (outward) down the pressure gradient. |
758 | 760 | 760 | The diaphragm eventually relaxes fully, so the decrease in volume stops. PA equilibrates with PB, and airflow ceases. The system is now ready for another inspiration phase. |
INSPIRATION
Contraction of the diaphragm alone, or contraction of both the diaphragm and the external intercostal muscles, produces quiet inspiration. As the diaphragm contracts, it descends, and this makes the thoracic cavity longer. Contraction of the external intercostal muscles pulls the anterior end of each rib up and out (Figure 27-6, A). This also elevates the attached sternum and enlarges the thorax from front to back and from side to side (Figure 27-6, B). In addition, contraction of the sternocleidomastoid, pectoralis minor, and serratus anterior muscles can aid in elevation of the sternum and rib cage during forceful inspiration.
As the size of the thorax increases, the intrapleural (intrathoracic) and alveolar pressure decreases (Boyle’s law) and inspiration occurs.
At the beginning of each inspiration, intrapleural pressure (PIP) is about 758 mmHg. Thus the PIP is about 2 mmHg less than atmospheric pressure (frequently written −2 mmHg). During normal quiet inspiration, PIP decreases further to 756 mmHg (−4 mmHg) or less. As the thorax enlarges, it pulls the lungs along with it because of cohesion between the moist pleura covering the lungs and the moist pleura lining the thorax. Thus the lungs expand and the pressure in their tubes and alveoli necessarily decreases. Alveolar pressure decreases from an atmospheric level to a subatmospheric level—typically a drop of about 1 to 3 mmHg. The moment that alveolar pressure becomes less than atmospheric pressure, a pressure gradient exists between the atmosphere and the interior of the lungs. According to the primary principle of ventilation, air moves into the lungs. Eventually, enough air moves out of the lungs to establish a pressure equilibrium between the atmosphere and the alveoli—and the flow of air then stops.
The ability of the lungs and thorax to stretch, referred to as compliance, is essential to normal respiration. If the compliance of these structures is reduced by injury or disease, inspiration becomes difficult—or even impossible (Box 27-2 on p. 848).
For a summary of the mechanism of inspiration just described, see Figures 27-5 and 27-7.
EXPIRATION
Quiet expiration is ordinarily a passive process that begins when the pressure gradients that resulted in inspiration are reversed. The inspiratory muscles relax, causing a decrease in the size of the thorax and an increase in intrapleural pressure from about 754 mmHg (−6 mmHg) before expiration to about 756 mmHg (−4 mmHg) or more during respiration. It is important to understand that this pressure between the parietal and visceral pleura is always negative, that is, less than atmospheric pressure and less than alveolar pressure. The negative intrapleural pressure is required to overcome the so-called “collapse tendency of the lungs” caused by surface tension of the fluid lining the alveoli and the stretch of elastic fibers that are constantly attempting to recoil.
As alveolar pressure increases, a positive-pressure gradient is established from alveoli to atmosphere—and thus expiration occurs as air flows outward through the respiratory passageways. In forced expiration, contraction of the abdominal and internal intercostal muscles can increase alveolar pressure tremendously—creating a very large air pressure gradient.
The tendency of the thorax and lungs to return to their preinspiration volume is a physical phenomenon called elastic recoil. If a disease condition reduces the elasticity of pulmonary tissues, expirations must become forced even at rest.
Figures 27-5 and 27-8 summarize the mechanism of expiration just described.
Look for a moment at Figure 27-9. This figure shows the repeating respiratory cycle mapped out as changes in pressures and volumes. Note that intrapleural pressure is always less than alveolar pressure. This difference (PIP − PA) is called the transpulmonary pressure. Intrapleural pressure is always “negative” with respect to alveolar pressure. Transpulmonary pressure must be negative to maintain inflation of the lungs, as stated previously.
Pulmonary Volumes and Capacities
The volumes of air moved in and out of the lungs and the volume remaining in them are matters of great importance. They must be normal so that normal exchange of oxygen and carbon dioxide can occur between alveolar air and pulmonary capillary blood.
PULMONARY VOLUMES
An apparatus called a spirometer is used to measure the volume of air exchanged in breathing (Figure 27-10). A graphic recording of the changing pulmonary volumes observed during breathing is called a spirogram (Figure 27-11, A). The volume of air exhaled normally after a typical inspiration is termed tidal volume (TV). As you can see in Figure 27-11, the normal volume of tidal air for an adult at rest is approximately 500 ml (or 0.5 L).
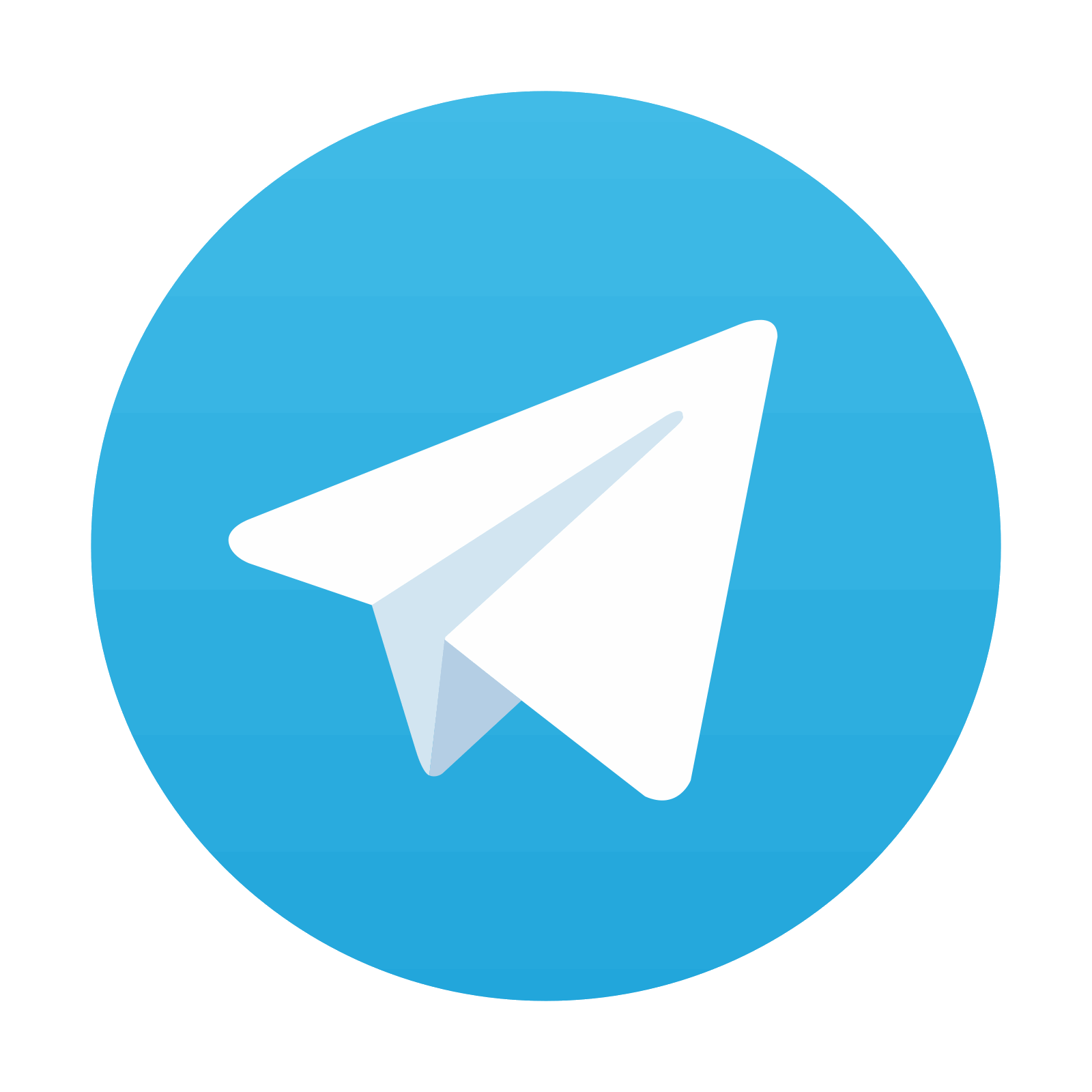
Stay updated, free articles. Join our Telegram channel
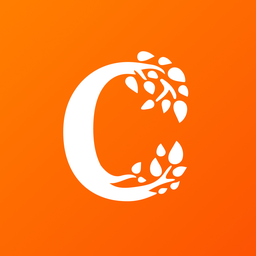
Full access? Get Clinical Tree
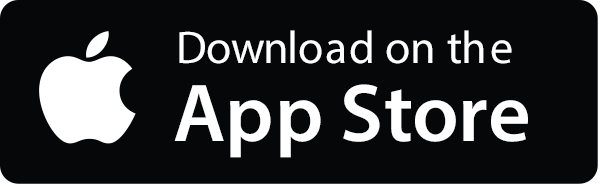
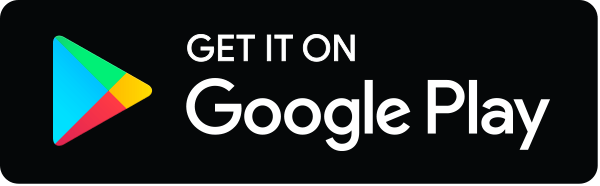