Overview of Epithelial Barriers |
CONTENTS
4.2.3 Regulation of Barrier Properties
4.3 Transport across Epithelia
4.3.2.1 Passive Transcellular Transport
4.3.2.2 Active Transcellular Transport
4.3.3.3 Receptor-Mediated Endocytosis
4.3.4 Physicochemical Properties of a Drug that Influence Epithelial Transport
4.3.4.1 Lipid Solubility and Partition Coefficient
4.3.4.2 Degree of Ionization and Drug pka
4.3.4.3 Molecular Weight and Molecular Volume
4.3.4.5 Lipinski’s Rule of Five
4.3.4.7 Implications of the Physicochemical Properties of a Drug for Epithelial Transport
4.4 Further Components of Epithelial Barriers
4.5 Properties of Distinct Epithelial Barriers
4.5.4.2 Trachea and Bronchioles
4.5.4.3 Respiratory Epithelium
4.5.5 Female Reproductive Tract
Epithelial barriers are essential for life. These are cellular structures established and maintained at interfaces that separate the internal environment of an organism from the external world. For example, epithelia cover the external surface of the body and line the gastrointestinal (GI), respiratory, and reproductive tracts. All epithelia present an asymmetric complement of surface components, intracellular organelles, and cytoskeletal arrangements that are organized in accordance with the external environment they experience. Most importantly, all epithelia must maintain the homeo-static conditions of the body. Thus, epithelial barriers must perform a number of critical functions that are distinct for specific internal/external interfaces. Some of the best-known functions performed by epithelia relate to their ability to restrict the casual movement of water and solutes, unidirectional movement of specific molecules, sense changes at their surface, and transmit that information into the body (e.g., neuroepithelial cells), as well as exclude and respond to pathogens, toxins, and innocuous agents (e.g., myoepithelial cells). As one might expect, epithelia of the body are under constant stress by external challenges; epithelial cells have a relatively rapid turnover rate typically in the range of a few days to weeks.
The goal of this chapter is to provide an overview of the characteristics and properties for the various types of epithelia present in the body of interest for drug delivery strategies and describe factors that influence drug transport across epithelia. While all epithelial surfaces have the potential for drug delivery, poor accessibility (salivary glands, pancreatic ducts, etc.) or difficulty in sustaining drug exposure (e.g., esophagus) can limit such opportunities. Additionally, particularly harsh conditions (gastric mucosa) can limit the types of drugs to be considered for delivery. A thick mucus covering or high enzymatic burden at an epithelial surface can also limit drug delivery opportunities. In this overview, we will not suggest that any one epithelium is the best site for drug delivery. Instead, we will focus on how the properties of each make them more or less suitable for particular classes of drugs and delivery strategies based upon the physicochemical properties of these drugs and delivery systems. We will also make an effort to compare and contrast the biological and physiological properties of various epithelia to provide a rationale for optimal alignment of drug delivery strategies at particular epithelial surfaces.
Epithelia are organized into one layer (simple epithelia), or more than one layer (stratified epithelia) of closely associated cells, that are supported by a basement membrane (Paulsson 1992). Interaction with the basement membrane provides the basis for an asymmetric epithelial architecture, allowing each cell to establish specialized surfaces that face the external world (apical surface) or the subepithelial environment of the body (basal surface) and take on characteristic squamous, cuboidal, or columnar shapes. The basement membrane is composed of tissue-specific isoforms of laminin and collagen (commonly type IV), as well as particular proteoglycans, proteins, and glucosamines that are organized in a layered arrangement. These acellular structures not only provide a mechanism of support but also separate epithelial cells from underlying connective tissue that contains blood capillaries and lymph vessels; oxygen and nutrients must diffuse from the underlying capillaries. Interactions between surface-expressed elements on epithelial cells and specific basement membrane components influence their behavior, providing the basis for intracellular signaling that affects adhesion, shape, migration, proliferation, and differentiation. Desmosomes as well as hemidesmosomes and focal contacts provide a common basis for epithelial cells to maintain a close association with other epithelial cells or the basement membrane, respectively. Loss of these close contacts can induce a specialized form of epithelial cell apoptosis known as anoikis.
Epithelial barriers come in several forms, with their structure being dependent upon their required functions (Pakurar and Bigbee 2005). For example, the skin is a multilayered epithelium where the cells proliferate while in contact with the basement membrane and transition into a nonviable form as they migrate away from the basal cell layer. The resulting stratified epithelium, with a superficial layer that becomes morphologically and biochemically modified through the process of cornification, provides a barrier that limits water loss and provides protection from environmental challenges. Similar stratification of epithelia is observed in the majority of the buccal cavity and female reproductive tract, except in these cases the surface cells do not undergo cornification. Like the skin, stratified epithelia of the mouth and vagina present a multilayer format of cells that function to limit casual uptake of materials following topical exposure. Epithelia of internal tissues, such as the respiratory tract and most of the GI tract, have less issues with abrasion compared to the skin, mouth, and vagina, and are organized to focus more on absorption and secretion events rather than protection. These epithelia form more delicate single layers or pseudostratified layers of cells. The plasma membrane of these single layers or pseudostratified layers of cells in epithelia is organized into two distinct regions, namely, apical and basolateral domains, as a result of apical–basal polarization processes.
The apical plasma membrane domain of simple epithelia faces the lumen of a tissue that directly communicates with the outside world, essentially equal to the epithelia of the skin. Although the cornified stratified squamous epithelium of the skin appears dramatically different from the simple columnar epithelium of the small intestine, all epithelia are structured to organize their functional properties in a polarized manner that is derived from specific interactions with a basement membrane present at their basal (inside the body) surface. One essential feature of this polarity is the communication between adjacent epithelial cells, which affects not only the apical to basal bias of epithelial cells but also their side-to-side (planar) arrangement: an organization that is essential for the control of epithelial cell proliferation. Lateral interactions between adjacent epithelial cells are facilitated by desmosomes, which bring cells in close approximation, and gap junctions (GJs) that directly connect the cytoplasm of two neighboring cells to enable the passage of ions and small molecules of <1 kDa.
While GJs and desmosomes promote intercellular communication, these structures are insufficient to establish apical to basal bias in epithelial cells since they do not form a barrier sufficient to restrict the movement of lipids and proteins between the apical and basal plasma membrane domains. The mixing of apical and basolateral domain plasma membrane components is restricted by an annulus of selected proteins and lipid structures known as the apical junctional complex (AJC). The AJC is formed by two structures, the adherens junction (AJ) and the tight junction (TJ), which bring two adjacent epithelial cells in extremely close approximation (Tsukita et al. 2001). These structures are organized in specific arrangement, are stabilized at the apical neck of polarized epithelial cells by a specialized annular ring of actin and myosin filaments, and are associated with an adjacent population of desmosomes (Figure 4.1).
There is a polarized distribution of lipids: the apical membrane is enriched in sphingolipids and cholesterol, while the basolateral membrane comprises mainly phospholipids (Figure 4.2).
AJ structures are important to maintain the integrity of the AJC and play a critical role in the regulation of epithelial cell proliferation. E-cadherin, the dominant AJ protein involved in establishing calcium-dependent cell–cell contacts, interacts with catenin proteins to signal AJ status. While AJ and TJ structures both form annular ring structures in polarized epithelial cells, it is the TJ that establishes the barrier that restricts lipid and protein movement between apical and basal plasma membrane domains. These membrane domains are organized by selected phosphorylation states of inositol lipids (Shewan et al. 2011). This restriction of plasma membrane component migration between apical and basal domains is referred to as the fence function of the TJ.
Due to the extremely close apposition of plasma membranes at the AJC, these structures also impede the movement of transport of water, electrolytes, and other small molecules between adjacent epithelial cells. This is different from the form of impediment established of the cornified layer of the skin. In simple epithelia, transmembrane proteins bring epithelial cells into such close approximation that it was initially suggested membranes of adjacent cells were fused (Farquhar and Palade 1963). Our current understanding of TJ structures is that they are composed of family members from three classes of transmembrane proteins:
• Claudins
• TJ–associated marvel proteins, which include the protein occludin
• An immunoglobulin superfamily that includes the junctional adhesion molecules (JAM) and the lipolysis-stimulated lipoprotein receptor
Like the AJ, TJs organize with scaffold proteins that interact selectively with regulatory factors as well as cytoskeletal elements (Figure 4.3).
Claudins are thought to play a major role in the regulation of the paracellular transport of ions and molecules. Their two extracellular loops form pores in the paracellular space and, depending on the claudins, bear a variable population of positively and negatively charged amino acids; different epithelia express 3–5 discrete forms of the 27 currently known claudin family members. In this way, each epithelium has distinct permselective properties to affect/control/regulate the paracellular transport of ions. Various components of the TJ interact with specific polarity complexes that function to establish and stabilize the different phosphorylation states of inositol lipids present in the apical and basal plasma membrane domains of polarized epithelia. Defects or mutations in specific TJ components can disrupt their ability to maintain normal fence and barrier function, which have been linked to a variety of diseases.
4.2.3 REGULATION OF BARRIER PROPERTIES
Epithelial cells that make up the various epithelial barriers of the body are continuously being replaced due to the senescence, growth, or repair of frank lesions. Thus, the barrier function of an epithelium is constantly changing at a microscopic scale but will appear relatively constant macroscopically. Simultaneously, biological events may be dynamically altering the barrier properties of an epithelium. For example, proinflammatory cytokines (e.g., tumor necrosis factor alpha and interferon gamma) can incite TJ disorganization, resulting in reduced epithelial barrier function. At sites of infection, white cells such as neutrophils will be drawn between adjacent epithelial cells. Their ability to access sites of infection requires the local dismantling of TJs to allow for efficient transmigration, an event that results in only a very minor and transient local barrier loss. More importantly, these observations show that simple epithelial barriers, such as those in the gut and lung, can dynamically open and close to allow transit of AJC structures or sufficiently for an entire cell to pass through.
Dynamic opening and closing of AJCs is achieved through mechanisms that control intracellular signaling processes associated with phosphorylation states of key cytoplasmic regulators of this complex organization of proteins (Dorfel and Huber 2012). An elaborate cohort of kinases and phosphatases functions to stabilize TJ and AJ proteins in a specific manner; the outcome of these activities is the steady-state condition of a “closed” format of these structures. Transient “opening” of these structures involves an adjustment of phosphorylation status of a discrete set of AJ and TJ elements. There are several regulatory pathways involved in controlling phosphorylation events associated with dynamic AJC opening; these pathways appear to have the ability to sense the requirement to open the AJC, in particular with the TJ, to allow molecules of less than 10 kDa in size, versus entire cells, to pass (Madara 1998).
4.3 TRANSPORT ACROSS EPITHELIA
As discussed earlier, the various epithelia of the body perform different functions based upon their location and thus have distinct properties related to the potential for molecules to move across them. For example, properties of the skin epithelia are dominated by the role of providing a barrier that limits dehydration, while the respiratory epithelium must protect the body from inhaled particulates while performing efficient gas exchange. These barrier functions must not preclude essential functions required of these epithelia such as protecting the rest of the body from radiation energy or efficiently exchanging gases for respiration. Also, as discussed earlier, each of the epithelia of the body must try to retain these properties during periods of growth and following tissue repair in order to maintain homeostasis. In the context of this chapter, we will describe some of the general properties and the dynamic nature of various epithelia of the body that restrict the transport of materials of pharmaceutical interest. These properties will relate to three general pathways across epithelial cells (Figure 4.4):
1. Paracellular: transport between adjacent cells
2. Transcellular: transport through individual cells, using pathways that access the cell cytoplasm
3. Endocytosis/transcytosis: uptake and transport through individual cells using vesicles that do not involve access to the cytosol
Based upon the surface area of epithelia, this potential space between adjacent epithelial cells represents only a fraction of the overall surface exposure to the external environment. Transport through the paracellular pathway is limited by the presence of TJs. These structures can be considered as a system of charge-selective small pores of approximately 4 Å and a second pathway created by larger discontinuities in the barrier that lack charge or size discrimination. This pore size translates to roughly compounds >200 Da being restricted from transporting across the epithelial barrier. There are, however, variations in TJ properties between different tissues, in particular related to the charge of the permeating molecule. As noted earlier, each epithelium or even the region within an epithelium (e.g., intestinal crypt versus villus tip) can express a different set of claudin proteins, each with its own sealing and charge-selective properties (Figure 4.5). Thus, the permeability and permselective properties of these epithelia can be distinct based upon the combination of expressed claudin proteins. In the following texts, we describe several exemplary epithelia to demonstrate these points.
Claudin-1, claudin-3, claudin-4, claudin-5, claudin-8, claudin-11, claudin-14, and claudin-19 each function to produce tight seals in an epithelium without establishing much permeability (Krause et al. 2008; Krug et al. 2012). For example, claudin-1–deficient mice die within 1 day of birth due to excessive transepidermal water through their skin. Claudin-2 forms channels permeable to small inorganic or organic cations and water. Claudin-10b is also cation selective but appears to produce smaller pores than that of claudin-2, since claudin-10b is not permeable to water and shows strong preference for Na+ over Li+ and K+ ions, whereas claudin-2 is not selective between these cations. Claudin-15 is also a channel for cations and is indirectly involved in glucose absorption through its permselection for Na+. Claudin-10a and claudin-17 are noted for their anion selectivity. The nature of this selectivity is dependent upon the other claudins present. For example, claudin-10a in Madin-Darby canine kidney (MDCK) II cells increases permeability for Cl− and NO3−, but only NO3−, in MDCK C7 cells. Based upon these properties, it is not surprising to find that claudin-2, claudin-10b, and claudin-15 are found in leaky, cation-selective TJs like those of the kidney and small intestine and that these epithelia have slightly larger than average paracellular pores of roughly 6.5 Å.
Efforts have been made to enhance the paracellular transport of a pharmaceutical agent through modulation of TJ function. Some of these approaches selectively disrupt extracellular components of TJ and AJ proteins (peptides that emulate or antibodies that bind to these domains); others act to disorganize the specific membrane environment associated with the AJC (e.g., chitosan and thiolated polymers, EDTA). Still others appear to nonselectively disrupt the organization of functional TJ structures, such as sodium caprate. It has been suggested that sodium caprate increases paracellular permeability through decreasing the expression of the TJ protein tricellulin and thereby disorganizing tricellular contacts. Additionally, some approaches have copied methods used by certain pathogens that use secreted enzymes working at the external surface of the cell, as well as manipulation of intracellular signaling mechanisms that affect either TJs or AJs. It is important to note that opening the paracellular route can be achieved indirectly by affecting AJs, an event that leads to destabilized cell–cell contacts that result in TJ disorganization. More information on these various pathways and mechanisms are described in the relevant chapters dealing with each specific route of epithelial delivery (i.e., Chapters 7 through 13, inclusive).
In general, however, one can assume certain aspects of the paracellular pathway following its opening by such an agent. For example, if a transport enhancer causes TJs to open with the retention of the normal complement of claudin proteins at the plasma membrane, then one could envision a channel that is larger than the normal TJ pores and with charge properties similar to the native TJ structure. In this way, larger molecules could transport across the epithelia, but there may be preference to those with particular surface charge properties. If, however, the enhancing agents result in claudin internalization by the epithelial cell, the resulting paracellular pathway should not have charge preferences related to claudin properties. Making the assumption that the material accesses the entire epithelial surface area, transport across epithelial barriers via the paracellular route can be approximated similarly to transport via the transcellular route/pathway.
The majority of pharmaceuticals that have been developed have the ability to readily transport across epithelia to enter the systemic circulation of the body. Such agents are relatively small (<400 Da in mass) and, as described in Section 4.3.4, have certain physicochemical properties that allow for them to partition into and out of the various lipid and aqueous compartments of epithelial cells in a nonselective manner. This allows them to migrate across an epithelium based upon a chemical potential gradient. The flux of such molecules crossing the epithelium has been modeled according to Fick’s first law of diffusion, which states that the rate of diffusion across a membrane is proportional to the difference in concentration on each side of the membrane:
where
dm/dt is the rate of diffusion across the membrane
D is the diffusion coefficient of the drug in the membrane
k is the partition coefficient of the drug into the membrane
h is the membrane thickness
A is the available surface area
ΔC is the concentration gradient, i.e., Co – Ci, where Co and Ci denote the drag concentrations on the outside and the inside of the membrane, respectively
When sink conditions occur, it ensures that a large concentration gradient is maintained throughout the absorption phase, thereby enhancing the driving force for absorption. The maintenance of sink conditions means that
and thus Equation 4.1 is reduced to
Substituting further into Equation 4.2 gives
where P, the permeability constant, is defined as Dk/h and has the units cm/s.
This can be simplified further to give
Equation 4.4 indicates that the rate of diffusion is proportional to drug concentration. K1 is a pseudorate constant and is dependent on the factors D, A, k, and h.
A further point here is that in order to reach the underlying blood capillaries to be absorbed, the drug must pass through at least two epithelial membrane barriers (the apical and basolateral epithelial cell membranes) and also the endothelial membrane of the capillaries. In some cases, for example, in stratified epithelia such as that found in the skin and buccal mucosa, the epithelial barrier comprises a number of cell layers rather than a single epithelial cell. Thus, the effective barrier to drug absorption is not diffusion across a single membrane as described earlier but diffusion across the entire epithelial and endothelial barrier, which may comprise several membranes and cells in series. The parameters ΔC, D, K, h, and A of Equation 4.1 refer to transport across the entire effective barrier, from the epithelial surface to the circulating blood, rather than simply the apical plasma membrane. However, it would appear that, generally, it is ultimately the apical plasma membrane that is rate-limiting for drug absorption. That is, if the drug can get across this barrier, it is likely to be able to move at a comparable rate across all other membrane barriers. Thus, in transcellular passive diffusion, the epithelium can be considered to act as a single lipophilic barrier for drug diffusion.
As described in the following texts, transcellular transport can be further divided into (1) passive processes, in which a drug moves down its concentration gradient using only its own kinetic energy, with no input of energy from the cell, and (2) active processes, which require cellular energy.
4.3.2.1 Passive Transcellular Transport
4.3.2.1.1 Simple Diffusion
As described earlier, in simple diffusion, drug molecules migrate across an epithelium based on their concentration gradient, and the rate of transport is given by Fick’s first law. The epithelia act as a passive element in the process, and the drug has the potential to equally flux in an apical to basal direction (absorption) or a basal to apical direction (secretion), based upon its chemical potential gradient. Transcellular diffusion is the mechanism by which most conventional drug molecules traverse the epithelial interface. The physicochemical properties of a drug that favor transcellular passive diffusion are described in Section 4.3.4.
4.3.2.1.2 Facilitated Diffusion
In facilitated diffusion, an integral membrane protein assists in the transport of a specific substance across the membrane (Figure 4.4b). In channel-mediated facilitated diffusion, specialized proteins can function as a membrane channel or pore, allowing the transport of ions and small molecules to penetrate the lipid bilayer. In some cases, these proteins transport inorganic ions (e.g., Na+, K+, Cl–); in other cases, they transport organic molecules that are too hydrophilic to readily pass through the plasma membrane, such as sugars and vitamins. Channel proteins could thus potentially mediate the passage of any drug molecule of the appropriate charge and size through the plasma membrane; however, in general, most small molecule drugs are too large for transport through these channels.
In carrier-mediated facilitated diffusion, a solute initially binds to a specific carrier (also known as a transporter) on the apical side of the membrane. The carrier then undergoes a conformational change to allow molecules to be transported across and released on the other side. This is still a passive process, requiring no cellular energy. The glucose transporter for the intestinal uptake of dietary glucose is a well-studied carrier-mediated facilitated diffusion transporter (Figure 4.4b). Other sugars (fructose and galactose), as well as vitamins (including thiamine, nicotinic acid, riboflavin, and vitamin B6), are also known to traverse the plasma membrane via this method.
4.3.2.2 Active Transcellular Transport
4.3.2.2.1 Primary Active Transport
In primary active transport, energy derived from hydrolysis of ATP changes the shape of a carrier protein, which “pumps” a substance across a plasma membrane, against its concentration gradient. The most prevalent pump in membranes is the Na+/K+ ATPase, a protein pump that expels Na+ from cells and brings K+ in (Figure 4.4b). Many amino acids and vitamins are pumped into the enterocytes of intestinal villi via direct primary active transport mechanisms, using ATP.
4.3.2.2.2 Secondary Active Transport
Secondary active transport utilizes the energy originally established by a primary active transport process. First, an ion gradient (e.g., Na+ or H+) is created by a primary active transport pump, driven by the hydrolysis of ATP. The energy stored in this primary ion gradient is subsequently used to drive a second substance across the membrane, against its own concentration gradient.
Secondary active transport utilizes a carrier protein in the membrane that simultaneously binds the initial ion that has been actively transported (e.g., Na+ or H+), as well as the second substance to be transported (e.g., glucose). This carrier protein then undergoes a conformational change so that both substances move across the membrane at the same time. A symporter moves two substances in the same direction, whereas antiporters move two substances in opposite directions (Figure 4.4b). In the intestines, glucose and galactose are transported into the enterocytes via secondary active transport that is coupled to the active transport of Na+. Some amino acids enter via Na+-dependent secondary active transporters; symporters have also been described for dipeptides and tripeptides traveling together with H+.
The implication for drug delivery is that a drug possessing structural similarities to a nutrient could potentially be taken into cells by specific transporters involved in the transport (passive or active) of that nutrient. For example, the drugs levodopa, methyldopa, and penicillin are all absorbed via various amino acid transporters. Serine and threonine derivatives of nitrogen mustard, which have been investigated for antitumor activity, are also absorbed by a carrier-mediated process. Furthermore, several successful approaches have also been described where drug molecules have been made into substrates for these carrier systems by chemical modifications that impart substrate-like properties.
A specialized form of transcellular transport involving vesicular trafficking is referred to as transcytosis. This is also an active process, requiring energy supplied by ATP. This process differs from transcellular transport in that the transporting drug never comes in contact with the cytosol of the epithelial cell and remains in vesicles that are contiguous with the external environment of the cell. Endocytosis at the apical surface of epithelial cells can occur through several mechanisms (Figure 4.4c).
Pinocytosis (bulk-phase endocytosis) is a nonspecific event that consists in plasma membrane invaginations that lead to the formation of intracellular vesicles filled with large volumes of extracellular fluid and engulfed molecules. No specific receptor proteins are involved and thus any solutes dissolved in the extracellular fluid are brought into the cells in a nonspecific manner. These vesicles travel in the cytosol and fuse with other vesicles but ultimately result in delivery to lysosomes.
Phagocytosis functions similarly with a terminal lysosome fate, but in this case, the plasma membrane invaginations are initiated to engulf cell debris, dust particles, or microorganisms. Specialized cells of the immune system, termed phagocytes, efficiently carry out phagocytosis; these cells include macrophages and neutrophils. Therefore, this transport mechanism is not usually relevant for drugs crossing epithelial barriers. However, a type of phagocytic uptake is carried out by specialized epithelial cells, known as M cells, in the Peyer’s patches of the GI tract (see also Chapter 7, Section 7.7). This transport process is thus being investigated for the oral delivery of drugs and vaccines, using nanoparticulate drug delivery systems (DDS). (Also, it is worth noting that for the parenteral route, the interactions of nanoparticulate DDS with phagocytic cells of the reticuloendothelial system have profound implications for the subsequent fate of these DDS in vivo, as described in Chapter 5, Section 5.3.)
4.3.3.3 Receptor-Mediated Endocytosis
Receptor-mediated endocytosis (RME) is distinct from pinocytosis and phagocytosis in that specific macromolecules are recognized via receptor binding and are brought into the cell in membrane vesicles that can be trafficked away from a lysosome fate. These ligand/receptor complexes are sometimes sorted to recycling endosomes with the ultimate fate of basolateral targeting. The uptake and transport of IgG antibodies and vitamin B12, and their release at the basal surface of epithelial cells, are two examples of RME that result in transcytosis desirable for drug delivery; these processes are receptor-mediated, energy-dependent, and highly regulated by the cell to minimize unwanted transport events. Conjugation of drug molecules (and DDS) with both IgG antibodies and vitamin B12 has been studied, in order to facilitate drug uptake via RME.
The use of endogenous pathways for the potential delivery of large hydrophilic drugs, such as proteins, into the body offers some exciting promise for the future. Such strategies are focused on simple epithelia, such as in the gut and lung, where these transcytosis pathways operate. Importantly, certain pathogens appear to hijack endogenous transcytosis pathways at these same simple epithelia to gain entry into a host. Some of these pathogen-targeted pathways have also been examined for the delivery of macromolecules.
4.3.4 PHYSICOCHEMICAL PROPERTIES OF A DRUG THAT INFLUENCE EPITHELIAL TRANSPORT
As described earlier, conventional drug molecules tend to be absorbed predominantly via transcellular passive diffusion across the epithelial interface, and the rate of diffusion is directly proportional to the concentration gradient across the epithelium (Equation 4.4). Diffusion is also critically dependent on the physicochemical properties of the drug, such as its lipid solubility, degree of ionization, size, and solubility (Florence and Attwood 2011); these factors are discussed here.
4.3.4.1 Lipid Solubility and Partition Coefficient
With respect to passive diffusion, the outer membrane of the epithelial cell may be regarded as a layer of lipid, surrounded on both sides by water (Figure 4.4b). Thus, for transport through the apical membrane, the drug must initially partition into the lipid membrane, according to its lipid/water partition coefficient, must then diffuse across the lipid phase at a rate determined by the concentration gradient, and finally, must be distributed out at the other side of the membrane, according to its lipid/water partition coefficient.
The degree to which a drug partitions into the lipid membrane is a measure of its lipid solubility and can be described by its oil/water equilibrium partition coefficient. This can be determined by adding the drug to a mixture of equal volumes of a lipophilic liquid (often octanol, but other solvents are also used) and water and shaking the mixture vigorously to promote partitioning of the drug into each phase. When equilibrium is attained, the phases are separated and assayed for drug. The partition coefficient (P) is given by
where
Coil is the concentration of drug in the oil phase
Cwater is the concentration of drug in the water phase
Often, the logarithm of the partition coefficient, (log P), of a compound is quoted. For a given drug, if log P = 0, there is equal distribution of the drug in both phases; if log P > 0, the drug is lipid soluble; and if log P < 0, the drug is water soluble.
A plot of percentage absorption versus log P yields a parabolic curve, with peak absorption occurring within a narrow optimal range of log P values. Values of log P that are too high (>6) are associated with poor transport characteristics. This is because highly lipidic drugs also have poor aqueous solubility, which limits bioavailability. Furthermore, if a drug is too lipophilic, it will tend to remain in the lipidic membrane and never partition out again into the underlying aqueous environment. Values of log P that are too low (<3) are also associated with poor permeation ability. Very polar compounds are not sufficiently lipophilic to be able to pass through lipid membrane barriers. If a drug molecule forms hydrogen bonds with water, desolvation and breaking of the hydrogen bonds is required, prior to partitioning into the apical membrane of the epithelial cell. If the number of hydrogen bonds between the drug and water is >10, too much energy is required and there will be minimal drug transport across the membrane. Drugs that are ionized will also demonstrate poor permeation ability, as described next.
4.3.4.2 Degree of Ionization and Drug pKa
Most drugs are weak electrolytes, existing in unionized and ionized forms in an aqueous environment. According to the pH-partition hypothesis, the unionized form of a drug is lipophilic and diffuses readily across cell membranes. Conversely, the ionized form is hydrophilic and thus cannot penetrate cell membranes easily. The degree of ionization (and therefore ability to cross a membrane) depends on both the pKa of the drug and the pH of the biological fluid in which it is dissolved.
The extent of ionization can be quantified by the Henderson–Hasselbalch equation (Box 4.1), which shows that for weak acids (with a pKa in the range 2.5–7.5), the unionized form of a weak acid predominates when the pH is lower than the pKa. Thus, weak acids will be predominantly unionized in the stomach, which favors their absorption in this region. In contrast, a very low percentage is unionized in the small intestine, which suggests unfavorable absorption for weak acids in this region. However, for weak bases (with a pKa in the range 5–11) the reverse is true: when the pH is lower than the pKa, the ionized form predominates. Thus, weak bases are poorly absorbed, if at all, in the stomach since they are largely ionized at low pH, but they are well absorbed in the small intestine, where they are unionized. Strong acids, such as cromoglycate, as well as strong bases, such as mecamylamine, are ionized throughout the GI tract and are therefore poorly absorbed.
Although the pH-partition hypothesis is useful, it must be viewed as an approximation because it does not adequately account for certain experimental observations. For example, most weak acids are well absorbed from the small intestine. Similarly, quaternary ammonium compounds are ionized at all pHs but are readily absorbed from the GI tract. These discrepancies arise because the pH-partition hypothesis does not take into account factors such as
• The large mucosal surface area of the small intestine can compensate for ionization effects.
• The relatively long residence time in the small intestine again can compensate for ionization effects.
• A local pH exists at the membrane surface that differs from the bulk pH of the GI lumen, due to the attraction of hydrogen ions to negatively-charged membrane components.
• Even the unionized form of a drug displays limited absorption.
• Charged drugs, such as quaternary ammonium compounds, may interact with organic ions of opposite charge, resulting in a neutral species, which is absorbable.
• Bulk transport of water from the gut lumen to the blood, or vice versa, can drag water-soluble molecules with it, resulting in a respective increase or decrease in the absorption of water-soluble drugs.
• Some drugs interact with the overlying mucus layer of the epithelium, which can affect the overall transport properties.
• Some drugs are absorbed via active pathways.
For ionizable drugs, log P is pH-dependent. Therefore, log D, the log distribution coefficient of the drug at different pHs, can be employed as an estimation and/or prediction of the absorptive potential at a particular pH value. The pH at which the log D is measured should therefore be reported, but in practice, values normally correspond to determinations carried out at a physiological pH of 7.4. The relationship between the observed overall partition coefficient and the distribution coefficient is given by the following equation:
where α is the degree of ionization of drug.
Although described here with respect to the oral route of drug delivery, it should be noted that all epithelial interfaces (buccal, sublingual, dermal, vaginal, pulmonary, nasal, etc.) show variations in local pH, and so these factors must be considered with respect to each particular route, absorption site, and drug moiety.
The Henderson–Hasselbalch Equation is
To illustrate the concept of the pH-partition hypothesis, consider the absorption of the weak electrolytes, aspirin, and codeine, from the GI tract.
WEAKLY ACIDIC DRUG ASPIRIN (pKa = 3.5)
In the stomach, assume the pH of the gastric fluid = 1.
The Henderson–Hasselbalch equation gives
Thus, at the low pH in the stomach, [HA] is for greater than [A–], i.e., the drug is predominantly unionized, that is, in the absorbable form.
In the intestine, assume the pH of the intestinal fluid = 6.5. The Henderson–Hasselbalch equation gives
Thus, at the high pH in the small intestine, [A–] is 1000 times greater than [HA], i.e., the drug is predominantly in the ionized, unabsorbable form.
WEAKLY BASIC DRUG CODEINE (pKa = 8)
In the stomach, assume the pH of the gastric fluid = 1.
The Henderson–Hasselbalch equation gives
Thus, at the low pH in the stomach, [BH+] is 107 greater than [B], i.e., the drug is predominantly ionized, that is, in the unabsorbable form.
In the intestine, assume the pH of the intestinal fluid is 6.5. The Henderson-Hasselbalch equation gives
Thus, at the high pH in the small intestine, the drug is much less ionized than in the stomach and is therefore more readily absorbed.
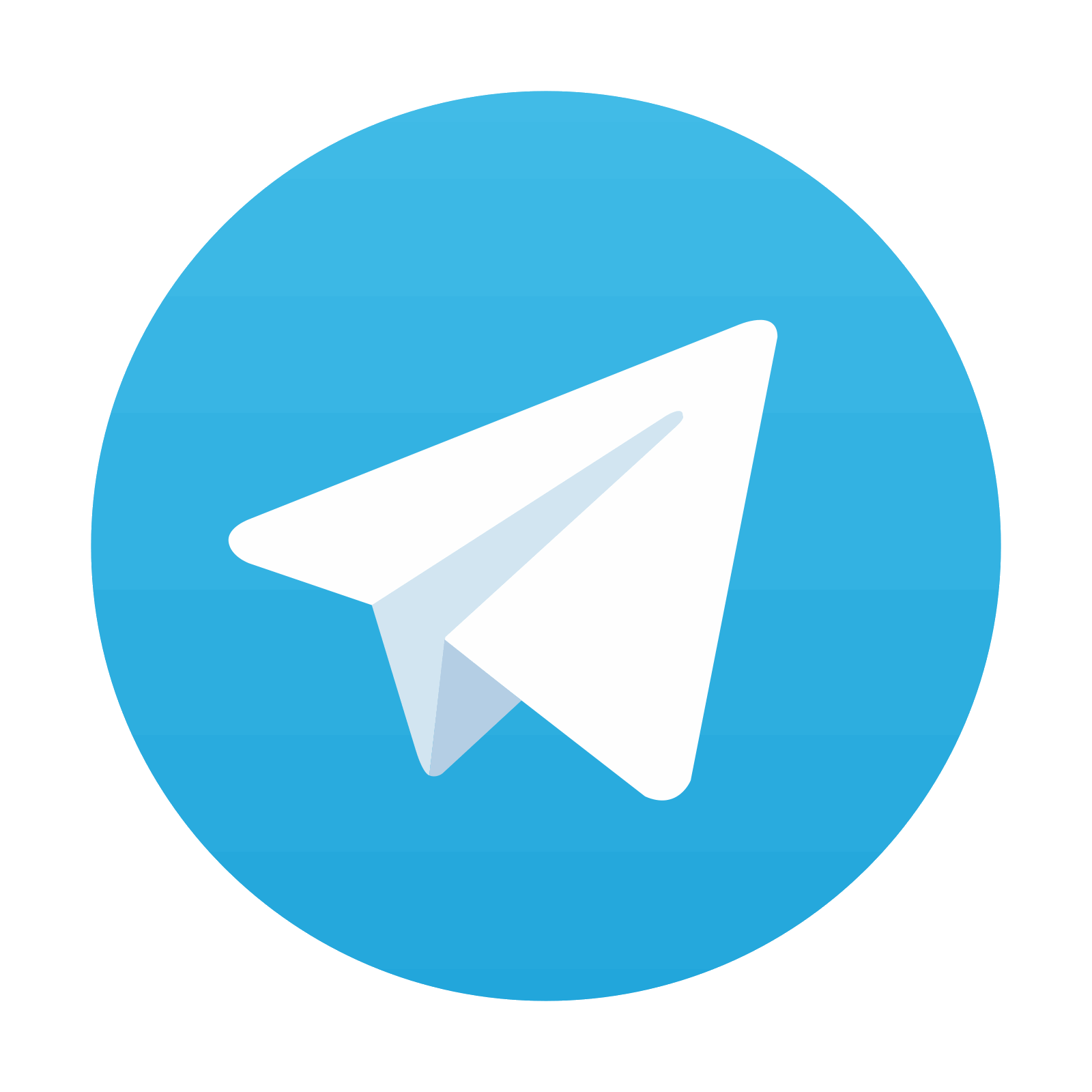
Stay updated, free articles. Join our Telegram channel
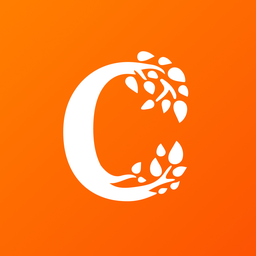
Full access? Get Clinical Tree
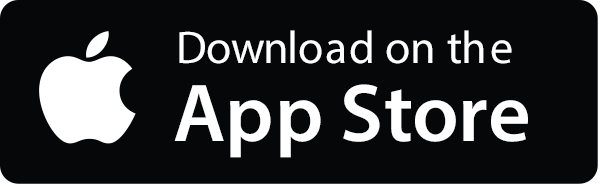
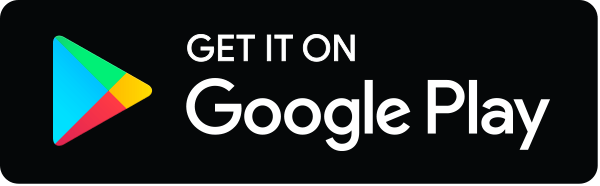