CONTENTS
7.2 Anatomy and Physiology of the GI Tract: Implications for Oral Drug Delivery
7.2.1 Advantages of the Oral Route for Drug Delivery
7.2.2 Limiting Factors for Oral Drug Delivery
7.2.2.4 P-Glycoprotein Efflux Pump
7.2.2.6 Variability in Conditions in the GI Tract
7.3 Mechanisms of Controlled Release for Oral Drug Delivery
7.3.1.1 Hydrophilic Swellable Matrix Systems
7.3.2 Reservoir Drug Delivery Systems
7.3.3 Orally Active Osmotic Pumps
7.3.4 Microelectronic Controlled Release
7.5 Regional Targeting for Oral Drug Delivery
7.5.1 Regional Targeting to the Stomach: Gastroretention
7.5.1.1 Floating Drug Delivery Systems
7.5.1.2 Size-Increasing Delivery Systems
7.5.2 Regional Targeting for Small Intestinal Delivery
7.5.3 Regional Targeting for Colonic Delivery
7.5.3.1 Microbially-Triggered Colonic Delivery
7.6 Improving Oral Bioavailability
7.6.1 Formulation Strategies to Improve Oral Bioavailability
7.6.1.4 Mucoadhesives and Mucolytics
7.6.2 Micro- and Nanoparticulate Drug Delivery Systems
7.6.3 Chemical Conjugation Approaches to Improving Oral Bioavailability
7.6.4 Systemic Delivery via the Colon
For patients, the oral route represents the easiest and most practical method of taking a drug; it is also usually the safest and least expensive route available. Commercially, as discussed in Chapter 21, the oral drug delivery market comprises the largest slice of the overall drug delivery market. However, the oral route is not without significant challenges. We begin our discussion with a consideration of the anatomy and physiology of the gastrointestinal (GI) tract, the implications therein for successful oral drug delivery, and the factors that must be taken into account in designing appropriate oral drug delivery systems (DDS). This is followed by a discussion on oral controlled-release (CR) technology, beginning with the most widely used and successful technology in oral CR: the hydrophilic matrix tablet. Dissolution- and/or diffusion-controlled matrix and reservoir constructs, osmotic-controlled devices, and microelectronic devices are also described. Drug targeting is discussed with respect to regional targeting within the GI tract: via gastroretention in the stomach and drug targeting to the small intestine (SI) and colon, respectively.
As described in Chapter 3 (Section 3.1.1), Class I molecules of the biopharmaceutical classification system (BCS) dissolve rapidly in the GI fluids and are highly permeable, so that they rapidly cross the epithelial barrier and are taken into the systemic circulation. It has proven much more difficult to achieve satisfactory oral absorption of larger, more polar active pharmaceutical ingredients (APIs), particularly those that are enzymatically labile. For example, the protein insulin, a 5808 Da protein that was introduced for the management of diabetes nearly 100 years ago (in 1923 by Lilly), is still not delivered via the oral route, despite intensive worldwide research efforts. Other poorly bioavailable APIs include polypeptides, proteins, and nucleotide-based drugs: the new “biologics” arising from advances in biotechnology and genomics. Currently, these entities must be delivered parenterally. Their successful oral administration offers many tantalizing rewards, including increased patient acceptance and compliance, reduced costs because sterile manufacturing and specialized health-care professionals are not required, expanded markets for drugs that could become major products if converted from daily or weekly injections, the potential for life cycle management of older injectable products, and the possibility of new intellectual property for oral systems with such molecules.
Strategies directed at enhancing oral bioavailability are considered from a formulation perspective via the use of excipients, including absorption enhancers (AEs), enzyme inhibitors (EIs), and solubilizers. Nanoparticulate DDS are described, as well as chemical conjugation approaches and the possibility of systemic delivery via the colon. Finally, the potential of the oral route for vaccination and the induction of mucosal immunity are discussed.
7.2 ANATOMY AND PHYSIOLOGY OF THE GI TRACT: IMPLICATIONS FOR ORAL DRUG DELIVERY
The anatomy of the GI tract is shown in Figure 7.1. It comprises a continuous tube of about 4.5 m in length in its normal contractile state, which extends from the mouth to the anus (Tortora and Derrickson 2013). It includes the organs of the mouth, most of the pharynx, the esophagus, the stomach, the SI (which in turn is divided into the duodenum, jejunum, and ileum) and the large intestine (which includes the ascending, transverse, descending, and sigmoid colon) (Figure 7.1). Secretions from accessory structures (including the salivary glands, liver, gallbladder, and pancreas) flow into the GI lumen via ducts and aid in the enzymatic breakdown of both food and drug molecules. A full description of the epithelium lining the GI tract is given in Chapter 4 (Section 4.5.3).
The mouth and drug delivery in the oral cavity (buccal and sublingual routes) is the subject of Chapter 8, and is not discussed further here. The stomach, a saclike chamber for the storage and digestion of ingested food, affords a hostile environment for many drug molecules, being both strongly acidic (with a pH in fasting conditions between 0.8 and 2), as well as containing many degrading enzymes (renin, pepsin, amylases). While the stomach does not comprise absorbing epithelia particularly appropriate for systemic administration, it is a target for retained local therapies as described in Section 7.5.1. The SI is concerned with completing the process of digestion started in the stomach, principally via the action of pancreatic enzymes secreted into the duodenal lumen. Bile salts, produced in the liver and stored in the gallbladder, aid in fat digestion and absorption. As described in Chapter 4 (Section 4.5.3), a number of structural features in the SI greatly increase the surface area available for absorption, (the presence of villi [see also Chapter 4, Figure 4.8], microvilli, plicae circulares, and intestinal glands) making this area of crucial importance to the absorption of digested nutrients and ingested drug molecules. The final stage of digestion occurs in the colon, through bacterial action. The colonic lumen is the home of 1012 FU/mL bacteria: this unique feature can be exploited for targeting to this region, as described in Section 7.5.3.
FIGURE 7.1 The gastrointestinal tract and (arrow) the accessory structures of the small intestine.
7.2.1 ADVANTAGES OF THE ORAL ROUTE FOR DRUG DELIVERY
The oral route offers many advantages for drug delivery, including the following:
1. An expanded surface area: The surface area of the SI in humans is approximately 200 m2, larger than the surface area of a tennis court and considerably larger than the surface area of other transmucosal routes such as the nose or oral cavity.
2. Absorptive epithelium: The specialized columnar epithelium of the GI tract is highly conducive to the rapid absorption of drugs of the appropriate physicochemical characteristics (see also Chapter 4, Section 4.5.3).
3. Rich blood supply: The highly vascular surface of the GI mucosa ensures rapid absorption and onset of action, as well as the maintenance of sink conditions.
4. Acceptability and compliance: The oral route allows for self-administration and is noninvasive. For patients, swallowing a pill or other oral DDS is simple, fast, and convenient. In comparison to all other possible routes of drug delivery, peroral drug administration demonstrates the highest patient acceptability and compliance. Compliance can be further improved by using CR dosage forms, which can convert three or four times daily-dosage regimens into much simpler, minimal-dosing schedules.
5. Amenable to CR and targeted delivery: The oral route is amenable to a wide range of drug release profiles, including immediate release (IR), delayed release (DR), sustained release (SR), CR, and pulsatile release (PR). Drug targeting to different regions of the GI tract is feasible, as is cell-specific targeting, albeit to a lesser degree.
7.2.2 LIMITING FACTORS FOR ORAL DRUG DELIVERY
A considerable number of factors in the GI tract may also limit oral drug delivery; these factors are considered here.
Transport across epithelial barriers is discussed in detail Chapter 4 (Section 4.3). To summarize here with respect to the oral route, paracellular transport is, under normal conditions, severely restricted by the presence of epithelial tight junctions between adjacent enterocytes and so is confined to small hydrophilic molecules, such as simple sugars like mannitol. Transcellular passive diffusion is usually the predominant pathway for low molecular weight, lipophilic drug molecules. The rate of absorption is governed by Fick’s law and is determined by the physicochemical properties of the drug, as well as the concentration gradient across the cells. The physicochemical properties of the drug that favor epithelial transport via transcellular passive diffusion have been described in detail in Chapter 4 and are summarized here:
• Molecular weight: Oral absorption is optimal for drugs with a molecular weight less than 500 Da.
• Lipid solubility and partition coefficient: The drug must be sufficiently lipid soluble to partition into the lipid bilayers of the plasma membrane of the enterocytes. Ionized, polar molecules have poor lipid solubility and thus exhibit poor transcellular transit. The optimal log P value for passive transcellular permeation is in the range of –0.4 to 3.5.
• Solubility: The drug must be soluble in the GI fluids for transcellular permeation. A major challenge of oral drug delivery is to increase the water solubility of poorly soluble BCS Class II and IV drugs. Strategies to increase drug solubility are discussed in Chapter 3.
• Drug pKa: This will affect the charge on weak acid and weak bases, as predicted by the Henderson–Hasselbalch equation (see Chapter 4, Box 4.1). The extent of ionization of a drug molecule varies according to the prevailing pH; it affects both solubility (ionized drugs are more soluble) and transepithelial permeability (unionized drugs are more permeable) properties.
• Chemical stability: The GI tract carries out biochemical breakdown of structurally complex foodstuffs of the diet into smaller, absorbable units. An API must be able to withstand the onslaught of digestive enzyme activity directed at foodstuffs. Acid- and base-labile drugs may be vulnerable to degradation, depending on the prevailing pH of the GI region.
A further mechanism of transcellular absorption is via carrier-mediated transport. A wide variety of nutrient carriers (including amino acid transporters, oligopeptide transporters, glucose transporters, lactic acid transporters, monocarboxylic acid transporters, phosphate transporters and bile acid transporters) exist on the luminal membrane of the enterocytes and serve as transporters to facilitate the absorption of digested foodstuffs by the intestine, using either active or passive mechanisms (see also Chapter 4, Figure 4.4). A drug molecule that possesses a similar structure to a nutrient may thus be transported by the nutrient carrier. A drug molecule may also be conjugated to a carrier substrate, such as a vitamin or sugar, so that the drug–substrate conjugate can be transported. However, many of these transport carriers are saturable, thereby limiting the amount of drug absorption possible. Competition between the drug and nutrient substrate may occur, with the concomitant risk of impaired nutrient absorption. Furthermore, nutrient transporters have evolved for the absorption of the digested products of protein nutrients, i.e., amino acids and di- and tripeptides, so that most peptide and protein drugs are too large for transporter uptake.
Enzymatic degradation occurs along the GI tract, beginning with the action of salivary amylase in the mouth. Pepsin and gastric lipase are found in the stomach. Enzymatic degradation in the SI is principally due to digestive enzymes secreted by the pancreas into the duodenal lumen (Figure 7.1). These pancreatic enzymes are capable of digesting all three categories of foodstuffs: (1) proteins, via serine proteases including trypsin, chymotrypsin, elastase, and procarboxypeptidases; (2) carbohydrates, via pancreatic amylase; (3) fats, via pancreatic lipase; and (4) nucleic acids, via pancreatic ribonuclease and deoxyribonuclease.
Drugs that structurally resemble nutrients, such as polypeptides, proteins, and DNA-based therapies, are highly susceptible to pancreatic enzyme degradation. Further enzymatic breakdown is carried out via the membrane-bound enzymes of the brush border of the enterocytes, which include disaccharidases and aminopeptidases. Enzymatic degradation in the enterocytes can occur either within the cell cytoplasm or within cellular lysosomes. In the large intestine, colonic microflora may also be involved in presystemic drug metabolism. Finally, venous blood draining from the digestive tract is first carried, via the hepatic portal vein, to the liver. An API absorbed from the GI tract may be metabolized by this first-pass effect (i.e., pre-systemic hepatic metabolism), prior to reaching the systemic circulation.
GI mucus comprises a complex mucus layer that coats the epithelium, protecting the GI tract. Several hundred microns thick, it presents a physical barrier that hinders the diffusion of drug molecules to the absorbing surface. A drug may also bind with the mucus layer, via hydrogen bonding, covalent interactions, etc. As mucus is alkaline, it may also affect the ionization (and by extension, absorption) properties of the API.
7.2.2.4 P-Glycoprotein Efflux Pump
This 1280 amino acid, 170 kDa protein functions as an energy dependent, drug efflux pump at the apical surface of cells. There is a high level of expression of P-glycoprotein (P-gp) in the epithelial cells of the SI. Substrate molecules that diffuse into enterocytes can be extracted directly back to the lumen via the pump. Thus, P-gp can play an important role in limiting the oral bioavailability of certain drugs, e.g., many anticancer drugs including vincristine and taxol are substrates of P-gp and not optimally available by the oral route.
The pH along the GI tract ranges from acidic to basic, with values ranging from 1 to 3 in the stomach, 4 to 5 in the duodenum, 5 to 6 in the jejunum, 6 to 7 in the ileum, and 7 to 8 in the colon. The pH of the fluids along the GI tract plays a critical role in the dissolution, solubilization, and intestinal permeability of ionizable drugs (see also Chapter 4, Box 4.1). Furthermore, acid- or base-labile drugs are susceptible to pH-mediated breakdown. For example, the extreme acidity possible in the stomach can rapidly degrade many peptide- and protein-based drugs, as well as drugs such as erythromycin, penicillin, and omeprazole.
7.2.2.6 Variability in Conditions in the GI Tract
The GI tract is characterized by a wide variability in the conditions that can exist along its length, which can affect consistency and reproducibility via this route. The large variation in pH along the GI tract has already been described. Another source of variability is the intestinal transit time. GI motility propels the luminal contents forward through the digestive tract, so that typically, small intestinal transit time is between 2 and 4 hours, although large variations are possible. Optimal drug absorption typically occurs in the upper jejunal region of the SI. A relatively short intestinal transit time can limit the effective contact time of an API with the absorbing surface and may thus compromise drug absorption. Posture, age, sleep, body mass index, physical activity, the presence or absence of food and fluid, overall diet, and disease states are all factors that can affect intestinal transit times. Similarly, there is a large inter- and intrasubject variation in the rate of gastric emptying, which can range from approximately 30 minutes to several hours.
Food in the GI lumen generally results in less efficient drug absorption due to a number of mechanisms. By slowing down gastric emptying, molecules unstable in gastric fluids are rendered more susceptible to metabolism. Food also provides a rather viscous environment, which may retard drug dissolution, as well as drug diffusion to the absorptive surface. Certain drugs may bind to food constituents forming a nonabsorbable complex (e.g., the chelation of tetracycline antibiotics with calcium ions in milk). GI fluids are secreted in response to food: enzymes present in the fluids may deactivate a drug moiety. Bile salts, secreted in order to aid in the emul-sification and absorption of lipids, may also destabilize lipophilic drugs and lipid-based drug carriers, such as liposomes and emulsions. The secretion and activity of bile salts exhibits high intra- and intervariability, making it difficult to anticipate these effects. Food constituents may also compete with drugs for carrier-mediated absorption mechanisms. A classic example is the breakdown products of dietary proteins, which can compete with L-dopa for oral uptake via an active transport mechanism. Further variability in the GI tract is afforded by individual subject variations such as gender, race, age, and disease state.
7.3 MECHANISMS OF CONTROLLED RELEASE FOR ORAL DRUG DELIVERY
Conventional oral dosage forms (solutions, suspensions, capsules, and tablets) feature immediate release (IR) of the API in the GI lumen, allowing the drug to dissolve in the GI contents and subsequently to be absorbed across the epithelium. Such dosage forms are useful when a rapid onset of action is required. There are many other types of drug release profile possible, as defined in Chapter 2 (Section 2.1), including sustained release (SR), controlled release (CR), modified release (MR), delayed release (DR), pulsatile release (PR), enteric release (ER) and stimuli-sensitive release (SSR). However, it should be remembered that the situation in vivo is highly complex, variable, and difficult to precisely ascertain. In particular, it can be difficult to differentiate accurately between SR and CR delivery systems and in this chapter, the terms CR and SR are used interchangeably.
The first commercial oral SR formulations date back to the early 1950s, with the introduction of the Spansule® system by Smith, Kline & French Ltd. Each Spansule® consisted of a capsule, loaded with hundreds of beads. Each bead comprised a drug core, surrounded by a waxy coating. Upon ingestion, the outer capsule rapidly disintegrated, liberating the coated beads. SR was facilitated by the gradual dissolution of the waxy coating as the beads traveled down the GI tract. The SR profile could be manipulated by mixing pellet populations of different coating thicknesses (see Chapter 1, Figure 1.2). Since the 1950s, oral SR technologies have become increasingly sophisticated (Omidian et al. 2011), to include a wide range of specialist technologies, as described next.
In a matrix system, the API is dispersed within a polymer network that retards drug release. Drug release from “swell-and-gel” hydrophilic matrices, hydrophobic matrices, and geometric matrices are described here.
7.3.1.1 Hydrophilic Swellable Matrix Systems
More than 75% of all oral CR delivery products currently marketed are based on hydrophilic matrix tablets. Their widespread use can be attributed to their simplicity, robustness, and versatility. Their method of manufacture is similar to that for conventional tablet formulation (i.e., granulation, blending, compression, and coating), thus established and cost-effective process technology is used, without the need for specialist technology or equipment (Rajabi-Siahboomi et al. 2008).
In hydrophilic matrices, the API is dispersed within a water-swellable polymer, known as a glassy hydrogel. On ingestion, aqueous fluids penetrate the matrix, lowering the glass transition temperature of the polymer, so that it converts from the glassy state to the rubbery state. The polymer chains hydrate, relax, and swell, thereby forming an outer, rubbery, pseudo-gel surface layer (Figure 7.2). In this “swell-and-gel” system, the mechanism of CR is via drug diffusion: the drug dissolves in the incoming fluids and then diffuses out through the viscous, swollen, polymer gel network (Rajabi-Siahboomi et al. 2008; Siepmann and Siepmann 2008; Liu et al. 2011).
Three distinct regions exist, which are defined within the matrix system during drug release (Siepmann and Siepmann 2008; Tu et al. 2010):
• Zone 1: The innermost area, where the polymer is in the dry, glassy state. The polymer is nonswollen. The drug is present as undissolved drug particles.
• Zone 2: A relatively thin layer, where the polymer chains are undergoing hydration and relaxation from the glassy state. The polymer is beginning to swell and disentangle; there is a mixture of both dissolved and dispersed drug.
• Zone 3: The outermost area, where the polymer is completely swollen, due to maximal elongation of the polymer chains. In this region, the drug is dissolved; the polymer per se may also be undergoing erosion and dissolution.
FIGURE 7.2 Hydrophilic “swell and gel” matrix drug release.
Three fronts can be defined, at the corresponding interface of each zone (Figure 7.2): (1) the swelling front, where nonswollen polymer is starting to swell with water; (2) the diffusion front, where the disentangling polymer molecules start to maximally extend; and (3) the erosion front, which exists at the interface between the GI fluids and the polymer.
The rate of drug release is complex and is determined by the rate of drug diffusion through the gel barrier, which in turn is affected by many factors, including drug solubility and the degree of polymer hydration, swelling, and porosity. In many cases, erosion constitutes a possible further release mechanism: the swollen hydrated chains display weakened mechanical properties, which may cause the hydrated polymer to break away from the system. This polymer disintegration leads to further dispersion and dissolution of the drug. Polymer dissolution may also occur, thereby facilitating the formation of pores and channels in the system and further modulating release rates.
From Figure 7.2, it can be seen that as water diffuses into the matrix, the drug diffuses out. Drug in the outer boundary will leave first, resulting in the formation of an outer layer of drug-free matrix. As more drug diffuses out, the layer of drug-free matrix (the depletion zone) becomes larger (see also Chapter 14, Figure 14.11). Therefore, the drug has an increasingly longer distance to travel before it is released from the system, resulting in a decreased amount of drug released over time (see also Chapter 2, Section 2.4.2.2). Although there has been considerable research effort directed toward developing zero-order, constant release, oral delivery systems, in the belief that “flatter is better,” in practice, this is not always necessary. A gradual tailing off in the release profile is perfectly acceptable, as long as the drug is maintained within the therapeutic range (i.e., below the toxic concentration and above the minimum effective concentration) for an extended period. While plasma levels are within the therapeutic range, there is enough drug present to produce a therapeutic response—it is not therapeutically necessary to have constant drug release.
Product (Active) | Company | Indication |
Tegretol® XR (carbamazepine) | Novartis | Anticonvulsant |
Ambien® CR (Zolpidem) | Sanofi | Sedative |
Xanax® XR (alprazolam) | Pfizer | Anxiolytic |
Glucophage® XR (metformin) | BMS | Antihyperglycemic |
Wellbutrin® XR (bupropion) | Valeant | Antidepressant |
Keppra® XR (levetiracetam) | UCB | Anticonvulsant |
Advicor® (niacin XR and lovastatin) | Abbott | Anticholesterol |
Simcor® (simvastatin and niacin) | Abbott | Anticholesterol |
Niaspan® (niacin) | AbbVie | Antihyperlipidemic |
Intuniv® (guanfacine) | Shire Pharma | ADHD |
Most water-swellable polymers used in hydrophilic matrices are based on cellulose and include hydroxypropyl methylcellulose (HPMC) and methylcellulose (MC). HPMC is by far the most widely used, forming a component of many commercially available preparations (Table 7.1). HPMC offers many advantages for hydrophilic matrix formulation, including fast and uniform gel formation, which is crucial to protect the matrix from disintegration and premature drug release (Rajabi-Siahboomi et al. 2008). The gel formed by HPMC is strong and viscous, which provides the necessary diffusional barrier to control drug release. Different chemistries (degree of methoxyl and hydroxypropyl substitution) and viscosities of HPMC allow customization of the desired release profile. It may also be combined with other polymers to offer further flexibility and control of release. Combinations with water-insoluble ethyl cellulose (EC) polymers can be used to delay release rates, whereas combinations with soluble polyvinylpyrrolidone (PVP), and poly(ethylene oxide) (PEO) polymers can be used to enhance solubility and dissolution rate. Incorporation of the anionic polymer sodium carboxymethylcellulose promotes drug release via erosion-mediated mechanisms.
Alternatives to cellulose as the matrix material release include xanthan and locust bean gums, as used in the Timerx® system. This technology is used in Opana® ER for the extended release of the opiate analgesic, oxymorphone hydrochloride. Contramid® uses a chemically and physically modified high-amylose cornstarch: the release rate can be customized by altering the degree of starch cross-linking. Commercial preparations include once-daily Ryzolt® (tramadol) for pain management and Oleptro® (trazodone) for depression.
Much less widely used perorally than their hydrophilic counterparts, hydrophobic matrices can be formed from water-insoluble polymers such as (1) EC; (2) PVC; (3) poly(methacrylates), e.g., the Eudragit® NE and NM series; and (4) fatty acids, alcohols, and waxes. The matrix can be either homogeneous, where the drug is partly dissolved and evenly distributed throughout the matrix, or porous, in which the matrix contains an additional soluble polymer, which dissolves quickly in the GI fluids, leaving pores in the release matrix to facilitate drug release. Although inert matrix technologies have been largely confined to parenteral implantable systems (see also Chapter 6), there has been recent interest in their use for peroral extended release, due to their ease of manufacturing and as an alternative to hydrophilic matrices. As per hydrophilic matrices, the rate of drug release typically tails-off with time. Hydrophobic matrices may be excreted intact (“exhausted ghosts”) in the stools following drug release in the GI tract.
FIGURE 7.3 Geomatrix™ technology.
In recent years, research has been directed toward modifying the drug release profile from matrix tablets, to try and convert the declining drug release rates typically obtained with matrix systems to a constant release rate over time. In the Geomatrix™ multilayered tablet, the geometry of the tablet is modified to enhance zero-order release (Conte et al. 1993; Moodley et al. 2012). The technology comprises an inner core layer, in which the drug is dispersed within a highly swellable hydrophilic polymer matrix (e.g., HPMC). The core is flanked by one or two impermeable, or semipermeable, polymeric coatings, applied to one, or both, bases of the core (Figure 7.3). On ingestion, the external flanking layers initially physically restrict the core layer from exposure to the aqueous fluids of the GI tract, thereby reducing the available surface area of the core for drug release. However as time progresses, the hydrophilic core swells, thereby increasing the exposed surface area available. This progressive increase in surface area with time compensates for the increasing path length, so that zero-order release can be achieved (Conte et al. 1993) (see also Chapter 1, Section 1.6).
Drug-specific customized release can be achieved by varying the number and type of flanking polymers and the core polymer characteristics. For example, zero-order release for the highly soluble drug, diltiazem, was achieved by using blocking polymers of hydrophobic EC on either side of the core. In contrast, low-viscosity hydrophilic HPMC polymers were required for zero-order release of the sparingly soluble calcium blocker nicardipine. Currently, the GeomatrixTM platform is used commercially to provide zero-order extended release in Paxil CR™ (paroxetine), Requip® (ropinirole), and Coruno® (molsidomine). The NSAID diclofenac (Diclofenac-ratiopharm®) is delivered in a biphasic profile with Geomatrix™: an initial burst release of the active drug followed by sustained release of the anti-inflammatory drug over several hours. More than one drug can be delivered with the system: Madopar® DR is a three-layered matrix tablet for the management of Parkinson’s disease, which releases a fraction of levodopa and benserazide within an hour and then further amounts of levodopa continuously over 6 hours. This is designed to have a steadying effect, minimizing the troubling “on–off” phenomenon that is seen with conventional Parkinson’s drug therapy.
7.3.2 RESERVOIR DRUG DELIVERY SYSTEMS
In reservoir DDS, a drug reservoir is surrounded by a polymeric coating, which functions as a ratecontrolling membrane (RCM; see also Chapter 1, Figure 1.3). Release can be controlled by modifying the coating composition, including the type and thickness of the coating polymer, the number of polymer layers, and the presence of excipients such as pore formers and fillers. Reservoir systems for oral drug delivery typically use water-insoluble polymers, in order to provide a constant diffusional barrier to drug release. Widely used polymers include EC, as well as polymers from the Eudragit® NE and NM series. Hydrophilic polymers such as HPMC, poly(vinyl alcohol) (PVA), and polyethylene glycol (PEG) can also be included as pore formers in the membrane.
Drug release from reservoir systems is based on diffusion of the drug through the coating membrane and will follow Fick’s laws of diffusion. If both the drug permeability and the drug concentration in the reservoir are constant, zero-order drug release will, in principle, be attained. In practice, this is not typically so—for example, water ingression on contact with the GI fluids may cause swelling of the polymer coating, which will affect coating thickness and the partition coefficient of the drug into the coating. Furthermore, as time proceeds after ingestion, the reservoir may become exhausted of drug. Zero-order release is further confounded by lag effects (an initial delay in drug release, as drug initially diffuses through the coating, before attaining equilibrium release conditions) and burst effects (an initial burst of release, which may occur if the drug has already diffused through the coating during storage). Nevertheless, although perfect zero-order release is not achieved, a “near-zero” release profile is possible.
Although “near-zero” release is possible, a number of disadvantages are also associated with reservoir DDS. First, there are manufacturing capability complications, as the knowledge, expertise, and equipment to apply specialist coatings are required. Second, there is the susceptibility of these systems to lag times and burst effects. Third, reservoir systems run the risk of dose dumping if the surrounding coat is damaged—tablets must carry a warning for patients not to crush or chew the tablet. Finally, as described earlier, if the polymer coating is not dissolved or otherwise degraded, the drug-voided shell will remain intact and be excreted by the patient. Many of these disadvantages can be overcome by using reservoir DDS as multiparticulate dosage forms, described in Section 7.4.
7.3.3 ORALLY ACTIVE OSMOTIC PUMPS
OROS™ (i.e., ORal OSmotic) technology uses osmotic pressure as the mechanism to control drug release from the delivery system. (Note: OROSTM was the original trademark of the Alza Corporation, however, the patent has expired and the term is now used generally, to describe oral osmotic release systems.) Importantly, the osmotic pressure of the GI fluid remains constant throughout the GI tract, regardless of the formulation used, or the surrounding environmental conditions. Thus, the extreme variability in conditions that characterizes the GI tract is circumvented, allowing for much more reliable and reproducible oral drug delivery.
Examples of OROS technology include the bilayer push–pull osmotic pump (PPOP) (see also Chapter 2, Section 2.5.1), as used in Glucotrol XL®, for the once-daily administration of glipizide, a blood-glucose-lowering drug for diabetic patients, as well as Procardia XL®, a CR formulation of nifedipine for the treatment of hypertension. Since their introduction by Pfizer in the 1980s, these two drugs have become top sellers in their respective market segments. Other highly successful examples of the PPOP include Ditropan XL® (oxybutynin) and Cardura XL® (doxazosin).
The Oros-Concerta® is a trilayer push–pull system for the delivery of methylphenidate in the treatment of ADHD in children (Rosen and Abribat 2005). Conventional IR preparations of methylphenidate necessitate twice daily dosing, with one of the doses required during school hours, which can be stigmatizing for affected children. To complicate matters further, tolerance to methylphenidate is associated with constant plasma levels of the drug. Oros-Concerta® is a trilayer tablet consisting of one push layer and two separate drug layers: (1) a lower layer, containing a high concentration of methylphenidate, and (2) an upper layer, with a lower drug concentration (Figure 7.4a). An outer IR layer of methylphenidate provides an initial quick onset of active. Then, as the osmotic mechanism begins to operate, drug is released via the orifice. The ascending concentration release profile overcomes the development of drug tolerance associated with constant plasma levels of methylphenidate (Rosen and Abribat 2005; Katzman and Sternat 2014).
The liquid OROS (L-Oros®) system provides for the osmotic-powered delivery of drugs in a liquid form, thus combining the advantages of CR with the high bioavailability of a liquid formulation (Rosen and Abribat 2005). A soft capsule, containing the liquid drug, is in turn surrounded by three further layers: a barrier layer, an osmotic engine layer, and a rate-controlling semipermeable membrane (Figure 7.4b). A delivery orifice is drilled through the outer three layers. When the osmotic engine expands, it compresses the soft gelatin capsule, pushing the drug formulation out through the delivery orifice. The barrier layer ensures the capsule is separated from the osmotic engine, thereby minimizing hydration of the capsule and mixing with the drug layer. The system is under study for the delivery of emulsions and microparticulate suspensions of highly insoluble drugs and polypeptides.
FIGURE 7.4 Oral osmotic drug delivery systems: (a) trilayer system and (b) liquid OROS system.
AndrxTM is an oral osmotic system in which the semipermeable membrane contains two laser-drilled exit ports, one on either side of the tablet, which facilitates an enhanced drug release from the system. Furthermore, greater dissemination of the drug through the GI lumen is expected on release, in comparison to a single exit point. The technology is used for the once-daily delivery of metformin (Fortamet®) and lovastatin (Altocor®).
Although a highly successful technology, OROS is not without drawbacks, chief among these being the high associated costs of manufacture—as very precise laser drilling is required, necessitating specialist knowledge and equipment. Dose dumping is again an issue, as the entire dose is contained in the drug reservoir. Also, the biologically inert components of the delivery system remain intact during GI transit and are eliminated in the stools as an insoluble shell. Finally, although theoretically perfect zero-order CR can be achieved with this system, it should be remembered that different segments of the GI tract typically demonstrate different absorption capacities. Optimal absorption usually occurs in the upper SI, and the drug absorption efficacy of the tissue typically decreases as the formulation transits along the intestine. Thus, even a zero-order drug release system such as OROS may not actually result in a constant drug concentration in the blood.
7.3.4 MICROELECTRONIC CONTROLLED RELEASE
Newer CR technologies are emerging based on microelectronics, which provide exceptionally precise control over drug release in the GI tract. For example, the InteliSite® Companion Capsule is a radio-frequency activated, nondisintegrating drug delivery device for oral drug delivery. A radiolabel included in the drug reservoir permits determination of the capsule location within a specific region of the GI tract via gamma scintigraphy. When the capsule reaches the desired location in the GI tract, the capsule contents are expelled via an active spring-loaded release mechanism. The subsequent release, degree of dispersion, and GI transit of the drug can be followed via radioimaging. The InteliSite® capsule is currently used in drug and formulation development, to precisely determine the rate and extent of drug absorption within specific regions of the GI tract.
The IntelliCap® system is a microelectronic drug delivery pill, comprising a drug reservoir and delivery pump, used in conjunction with an electronic microcontroller, wireless communicator, and sensors. The delivery device is mounted within a small pill-shaped capsule that is swallowed and moves through the GI tract. Within the GI tract, the capsule can take measurements of local pH and temperature. Analysis of the physiological measurements allows accurate localization of the capsule within a specific region of the GI tract. Once the desired site has been identified (e.g., the SI, colon), the capsule dispenses a metered dose of the therapeutic agent, according to a preprogrammed release profile and/or a command issued from an operator. The onboard electronics enable both extremely accurate, and highly adaptable, delivery release patterns.
Although the present generation of microelectronic oral delivery devices are for research purposes only, they do show great promise for the future provision of personalized care. These devices could facilitate the administration of the correct dose, at the correct time, in precisely the desired location in the GI tract. Drug release can be adjusted to the specific needs, and also physiological conditions, of each individual patient.
In multiparticulate dosage forms, the drug dose is divided out into multiple mini-unit dosage forms, most commonly using beads (“nonpareil” cores, 1–2 mm in diameter), which are then loaded into a gelatin capsule (Figure 7.5).
Multiparticulates can be used in combination with matrix and/or reservoir architecture, to achieve many different drug release options (Skalsky and Stegemann 2011). For example, the spheroidal oral drug absorption system (SODAS®, originally developed by Elan) is based on nonpareil cores, onto which a solution of the active drug is applied. The drug layer is covered by further layers of product-specific functional coating polymers, which provide the RCM (Figure 7.5). Drug release is controlled by diffusion across the membrane; membrane erosion, dissolution, and pore formation may also occur. In the SODAS® formulation for methylphenidate, each Ritalin® LA capsule contains two populations of beads: half of the dose as uncoated beads, for immediate effect; the remainder comprise drug cores layered with enteric-coated, delayed-release polymers for extended release. Numerous other products have been relaunched using the SODAS® technology, all of which permit once-daily dosing with minimal side effects compared to the conventional formulations, including Cardizem® LA (diltiazam), Avinza® (morphine), and Focalin® XR (dexmethylphenidate).
FIGURE 7.5 Multiparticulate drug delivery system for oral drug delivery.
Other examples illustrate the versatility of multiparticulate technology. Diffucaps® multiparticulates provide a delayed-onset release for the antihypertensive drug, propranolol. Innopran® XL is administered at bedtime, and the Diffucaps® polymer-coated beads ensure a delay in propranolol release for 4 hours, ensuring that maximum propanolol plasma levels occur in the early morning: the time when patients are most at risk of adverse cardiovascular events. A further example is Moxatag®, a once-daily extended release amoxicillin tablet formulation, based on the Pulsys™ multiparticulate platform. This technology utilizes different pellet populations, coated with enteric and CR polymers, to provide a triple-pulse delivery of amoxicillin along the GI tract. It is known that pulsatile antibiotic therapy can be a more effective and efficient modality than standard antibiotic regimens. Finally, as an alternative to using beads as the cores, Minitabs® are tiny (approximately 2 mm in diameter) cylindrical tablets, which can be coated with RCMs. This modality combines the simplicity of tablet formulation with the versatile CR capabilities of multiparticulates.
Multiparticulate dosage forms are technically more complicated, time consuming, and expensive to produce, requiring specialist know-how and equipment. However, they offer several advantages over their single-unit dosage counterparts:
1. CR: Multiparticulates can be used to provide a wide range of drug release patterns (e.g., IR, SR, CR, ER, delayed-onset release, pulsatile biphasic and triphasic release). The final capsule can be loaded with a mixture of different bead populations, thereby facilitating multiple release profiles and/or multiple active ingredients—all within a single dosage form.
2. Reduction in GI variability: Multiparticulates are not subject to the vagaries of gastric emptying. Gastric emptying is an “all-or-nothing” process, so that a single-dose solid dosage form such as a tablet is either all in the stomach or all in the duodenum. Due to the large inter- and intrasubject variation in the rate of gastric emptying, there can be extreme variability in the bioavailability with single-dose forms (SDFs). In contrast, when the gelatin capsule of a multiparticulate formulation dissolves in the stomach, hundreds of coated pellets are released, all small enough to pass through the pyloric sphincter, even if the sphincter is closed. Variability issues, such as whether the patient is in a fasted or fed state or the patient’s posture, are also minimized. Furthermore, the pellets are widely dispersed throughout the GI tract, which tend to reduce the effect of variations in GI motility, and GI pH, along the tract.
3. Avoid dose dumping: In a multiparticulate system, the dose is distributed among hundreds of mini-units. Many hundreds of pellets would have to fail before dose dumping could occur, making it a far less likely event than for a single unit Solid Dosage Form (SDF).
4. Convenience: Multiparticulates are more convenient for the patient, as the capsules can be opened and sprinkled onto food or beverages (“sprinkle dosing”), a particular advantage for pediatric and geriatric patients, who often experience difficulty swallowing tablets.
7.5 REGIONAL TARGETING FOR ORAL DRUG DELIVERY
Microelectronic-controlled drug release has been described in Section 7.3.4 and features oral capsules that, via remote signaling, can release their payload at the desired site in the GI tract; as such, they are capable of very precise regional targeting. Here, we describe other mechanisms to achieve regional drug targeting, to the stomach, SI and colon, respectively. Targeting oral vaccines to the Peyer’s patches (PP) is a further area of research and is described in Section 7.7.
7.5.1 REGIONAL TARGETING TO THE STOMACH: GASTRORETENTION
For drugs with a narrow absorption window, there is a risk that they may transit their optimal absorbing region too rapidly, limiting the extent of absorption possible. Gastroretention (GR) strategies retain a drug in the stomach for an extended period, allowing it to be slowly and gradually released, for subsequent optimal absorption in the SI. GR strategies are also useful for drugs absorbed via saturable transport mechanisms in the upper SI (e.g., L-dopa, gabapentin): a slow, extended presentation of the drug to its transporter minimizes the possibility of transporter saturation. GR devices can also be used to retain drugs in the stomach for local action (e.g., antacids, antibiotics to treat Helicobacter pylori for ulcer therapy, cytotoxics for gastric cancer) and for drugs that have poor solubility at small intestinal pH, e.g., cinnarizine.
To achieve successful GR, formidable physiological challenges must be overcome; in particular, the dosage form must be retained in the stomach during the very powerful housekeeper wave of the migrating motor complex. A wide variety of GR systems have been developed, using many different approaches (Streubal et al. 2006; Pawar et al. 2011). The principal approaches are described here and summarized in Figure 7.6.
7.5.1.1 Floating Drug Delivery Systems
In this approach, the DDS floats on top of the stomach contents, retarding transit to the pylorus and prolonging gastric residence time (Pawar et al. 2011; Prajapati et al. 2013). Obviously, sufficient fluid must be present in the stomach to support these systems. Buoyancy can be achieved in a number of ways (Figure 7.6a). Effervescent systems are based on the inclusion of bicarbonate, citrate, or tartrate and cause the release of CO2, which becomes partially entrapped in the dosage form and provides sufficient buoyancy for floatation. Cifran OD® uses a gas-generating system for the gastroretention of ciprofloxacin. Liquid Gaviscon® contains sodium alginate, which reacts with HCl in the stomach to form a thick, alginate, raftlike layer. HCl reacts with calcium carbonate and sodium hydrogencarbonate in the formulation, to form bubbles of CO2, which become trapped in the alginate raft, enabling it to float on the surface. The raft forms a strong physical barrier against reflux, while antacids in the formulation neutralize excess HCl.
A hydrodynamically balanced system (HBS) comprises a floating capsule, based on the use of gel-forming hydrocolloids, which then entrap air for buoyancy. The drug is mixed with swellable, gel-forming, hydrophilic polymers such as HPMC and then encapsulated. On ingestion, the capsule disintegrates and water starts to penetrate the system, causing swelling and hydration of the polymers. The air trapped by the swollen polymers allows the system to float. In addition, the gel layer provides diffusion-controlled sustained release. The HBS system is available commercially for the delivery of levodopa (Madopar® HBS), a drug that is absorbed by a saturable carrier system from the SI and thus benefits from a slower arrival at the SI. Madopar® shows less side effects and less “on–off” phenomenon than conventional formulations. Valrelease® also uses an HBS system for the sustained delivery of diazepam. Diazepam is preferentially absorbed in the stomach, due to its increased solubility in acidic pH, making it an ideal candidate for gastric retention.
Porous foam powder systems incorporate a highly porous, polypropylene foam powder in the formulation, to provide an inherent low density. Both single-unit systems and also floating microparticles have been shown to have excellent in vitro floating properties and a broad spectrum of drug release patterns.
7.5.1.2 Size-Increasing Delivery Systems
These DDS are initially small, to facilitate swallowing, but then they massively, and rapidly, increase in size in the stomach, so that the system becomes physically too large to pass through the pyloric sphincter. The products must not increase so much in size that they adversely affect GI function. An example of an expandable system is the Accordion Pill™, in which the drug, or combination of drugs, is loaded onto a polymeric film, which is then folded into an accordion-like shape and packed into a gelatin capsule (Figure 7.6b). After the capsule dissolves in the stomach, the accordion unfolds and is retained in the stomach for up to 12 hours. Drug is released from the expanded system via diffusion. A variety of other expandable systems are currently being investigated, which expand into various complex shapes in vivo. A major challenge with this approach is the difficulty in the manufacture of such complex constructs.
Superporous hydrogels are a class of hydrogels with, as the name implies, very large pore sizes (more than 100 μm, which contrasts with the nanometer size range of conventional hydrogels). The large pore size allows them to swell much faster, and to a greater extent, than conventional hydrogels, so that the systems become too large to pass through the pyloric sphincter (Figure 7.6c) (Chen et al. 2000). The addition of excipients such as croscarmellose sodium confers high mechanical strength on the system, enabling it to withstand the mechanical force of the peristaltic waves.
Acuform™ is a tablet that absorbs water from the gastric fluids and expands to 150% its original size. Patented swelling polymers facilitate the uptake of water, and the enlarged tablet is retained in the stomach for approximately 8–10 hours, while drug slowly diffuses out. Acuform™ technology is used for the once-daily oral administration of gabapentin (Gralise®), which is absorbed via the L-amino acid carrier in the jejunum. The IR formulation results in carrier saturation, hence multiple daily doses are required. Gralise® appears to cause less dizziness and sleepiness than the IR gabapentin formulation, so its advantages go beyond a simple convenient argument for a once-a-day tablet.
Mucoadhesive microparticles based on the hydrophilic, gel-forming polymer Carbopol® 934P have shown promise in preclinical studies for the GR of furosemide, amoxicillin, and riboflavin, compared to suspensions. Other microparticles and minitablets designed for gastric retention use chitosan, thiolated chitosan, and polycarbophil as the mucoadhesive polymer, with a range of payloads including tetracyclines and low-molecular-weight heparin (Figure 7.6d). Still, none have reached the market and there are major issues concerning high mucus turnover and weak gastric mucus adherence, leading to unpredictable retention times. Mucoadhesive polymers are discussed further in Section 7.6.1.4, as formulation excipients to improve oral bioavailability.
High-density systems incorporate excipients such as barium sulfate, titanium oxide, and iron salts, with a higher density than gastric acid (usually >3 g/cm2) to provide a sinking system (Figure 7.6e). Intuitively, this would seem to be an obvious approach, but there are technical difficulties in compacting high concentrations of active and preventing premature release: no system has yet reached the market.
Other research approaches include magnetic systems with external remote control of transit time. Ion-exchange resins can also be formulated to have gastroretentive properties. Resin beads can be entrapped with bicarbonate, an anionic drug is then attached to the resin and the composition is loaded into a semipermeable construct. When it reaches gastric juice, bicarbonate and chloride are exchanged, but the resulting gas is withheld in the membrane and causes the system to float. However, these approaches are still at the research stage.
Of interest is the development of gastroretentive intraruminal devices in large animal veterinary medicine. These large devices are designed to release antiparasitic molecules including ivermectin and morantel over the 9-month growing season in cattle and are designed to stay in the rumen after administration with a balling gun. Elegant intraruminal device strategies comprise principles of expansion, osmotic release, and rolled-up polymers with drug embedded in a porous matrix. Due to the different physiology between the monogastric human and ruminal stomachs, technology development has been parallel, but distinct, in respect of engineering designs, payloads, and clinical indications.
7.5.2 REGIONAL TARGETING FOR SMALL INTESTINAL DELIVERY
An enteric coating is designed to prevent drug release in the stomach; instead, release is delayed until the SI. Such coatings have a long history in pharmaceutical practice and have traditionally been used either to prevent the drug from causing stomach irritation (e.g., aspirin) or to prevent the acidic environment of the stomach from causing premature drug degradation (e.g., enteric coatings for penicillins). Many oral peptide formulations currently in clinical trials have enteric coatings designed to undergo initial dissolution at the pH of duodenal and jejunal fluid, thereby protecting the labile peptide from acid degradation in the stomach (see Section 7.6).
Almost all enteric coatings currently in use are synthetic, or semisynthetic, pH-sensitive polymers containing ionizable carboxylic groups. These remain unionized (and thus insoluble) in the acidic environment of the stomach, but become ionized (and thus soluble) in the less acidic conditions of the SI, thereby allowing the coating to dissolve and the drug to be released.
The most widely used group of polymers to achieve enteric coating are the poly(methacrylates), marketed as the Eudragit® L and Eudragit® S series. A wide range of copolymers within this series is available, allowing drug release across a wide range of pH conditions (Table 7.2). Cellulose-based derivatives are a further option, from either cellulose itself, such as cellulose acetate phthalate (CAP), or derivatives of the hydrophilic polymer HPMC, such as HPMC phthalate (HPMCP). Poly(vinyl) derivatives include poly(vinylacetate phthalate) (PVAP).
An extension of this idea is the use of pH-sensitive hydrogels, whereby the drug is trapped within a 3D hydrogel matrix that exhibits negligible swelling in the acidic conditions of the stomach, but drastically increased swelling in the alkaline environment of the SI, releasing drug (Figure 7.7). One of the most extensively studied of these systems comprises an anionic hydrogel comprising PEG grafted on poly(methacrylic acid) (Peppas et al. 2006).
TABLE 7.2
Threshold pH of Commonly Used Polymers for Enteric Coatings
Polymer | Threshold pH |
Eudragit® L100 | 6.0 |
Eudragit® S100 | 7.0 |
Eudragit® L30D | 5.6 |
Eudragit® FS30D | 6.8 |
Eudragit® L100-55 | 5.5 |
Polyvinyl acetate phthalate | 5.0 |
HPMCP | 4.5–4.8 |
HPMCP 50 | 5.2 |
HPMCP 55 | 5.4 |
Cellulose acetate trimellitate | 4.8 |
Cellulose acetate phthalate | 5.0 |
Abbreviation: HPMCP, hydroxypropyl methylcellulose phthalate.
FIGURE 7.7 pH-sensitive hydrogels for drug targeting to the small intestine.
7.5.3 REGIONAL TARGETING FOR COLONIC DELIVERY
Targeting drugs for local delivery to the colon is particularly appropriate for the topical treatment of inflammatory bowel disease (IBD), including ulcerative colitis (UC) and Crohn’s disease. First-line drug treatments for UC include (1) the anti-inflammatory drug, mesalazine (also known as 5-aminosalicylic acid [5-ASA]), which acts locally on the colonic mucosa to reduce inflammation, and (2) the anti-inflammatory corticosteroid, budesonide. A number of mechanisms are used to achieve drug targeting to the colon (Lichtenstein 2009; Omidian and Park 2010; Perrie and Rades 2012) and are summarized here:
1. Rectal delivery: A cohort of UC patients have restricted inflammation limited to the sigmoid colon, which is amenable to rectally delivered foams, liquid enemas, and suppositories. Foams incorporating mesalazine, or budesonide, are low in volume, adhesive, and viscous; they disperse as far as the descending colon. Scintigraphy studies with mesalazine reveal that foams can disperse more widely than enemas, for left-sided UC. Foams are well tolerated by patients and their local delivery means that systemic side effects are minimized.
2. pH-triggered colonic delivery: The most commonly used pH-dependent coating polymers for colonic targeting are Eudragit® L100 and Eudragit® S100, which dissolve at pH 6.0 and 7.0, respectively (Table 7.2). Drug release within this range can be tailored by using polymer combinations in various ratios. A number of commercial preparations based on pH-sensitive Eudragit-S resin coatings are available for the colonic delivery of mesalazine (e.g., Asacol®, Claversal®, and Mesasal®) and also budesonide (e.g., Entocort® and Budenofalk®).
3. Time-delay colonic delivery: The drug can be incorporated within a matrix- or reservoir-CR system comprising poorly soluble, slowly eroding polymers so that drug release is delayed until the colon is reached. A lag time of 5 hours is usually considered sufficient, given that gastric emptying takes about 2 hours and SI transit is about 3–4 hours. An example is the Pentasa® technology, based on the delayed release of mesalazine, using pellets coated with EC. More experimental time-delay systems include the following: (1) The Pulsincap™ uses a swellable hydrogel plug to initially seal an API within an insoluble capsule body. As the system passes through the GI tract, the hydrogel plug swells until eventually it is ejected from the capsule, thereby liberating the drug. (2) The OROS-CT™ is an oral osmotic device for colon targeting, based on as many as five to six push–pull osmotic units filled into a hard gelatin capsule (see Section 7.3.3). This allows for a lag time of 3–4 hours, thereby facilitating colonic targeting. However, neither of these approaches has been commercialized.
4. Combination approaches: Both the pH and time-delay methods outlined earlier are limited by the wide variability that exists in the GI tract with respect to GI pH and transit time. This results in a lack of consistency and reproducibility with these approaches. In order to improve colon-targeting efficacy, these strategies can be combined. Lialda® delivers mesalazine to the colon using a MultiMatrix (MMX®) technology. This sophisticated system uses an outer enteric layer of Eudragit-S film, which delays initial release of the active drug until the tablet is exposed to a pH of 7.0 or higher. As the coating disintegrates, intestinal fluids interact with an inner hydrophilic matrix, resulting in the formation of a viscous rate-controlling gel, which provides CR via diffusion. An additional lipophilic matrix, interspersed within the hydrophilic matrix, slows down the penetration of aqueous fluids into the tablet core, reducing the rate of drug dissolution, thereby prolonging drug release and therapeutic activity. The hydrophilic matrix may also adhere to the colonic mucosa, this bioadhesion can further facilitate targeted drug delivery.
Recent studies in man have revealed that nanoparticles have an inherent attraction to the inflamed colon in UC (Schmidt et al. 2013). The prospect of a combination approach, using pH-dependent coated capsules which release targeted nanoparticles for local delivery of payload in the inflamed colon, is therefore under intense research scrutiny. Research has focused on the use of polymeric nanoparticles based on poly(lactide-co-glycolide) (PLGA), although trimethylated chitosan, solid–lipid nanoparticles (SLNs), and lipid-based microemulsions are also being investigated.
7.5.3.1 Microbially-Triggered Colonic Delivery
Although bacteria are present throughout the GI tract, the colonic region is much more heavily colonized than the upper regions, due to the decreased peristalsis, presence of feces, and lower oxidation-reduction potentials of this region. These resident microflora are a highly specific environmental feature of the colon, thus making it a more selective approach for colon-targeting than methods such as pH-sensitivity or time-control.
One of the earliest prodrug approaches for local colonic delivery for the treatment of IBD was using sulphasalazine, which comprises a dimer of the anti-inflammatory 5-ASA (mesalazine) linked via an azo bond to a carrier molecule, sulphapyridine. The majority of the sulphasalazine reaches the colon intact, where the conjugate is cleaved by colonic microflora to the active metabolite, 5-ASA, and the by-product, sulphapyridine. Colon-specific targeting is achieved due to the absence of azoreductase activity in the upper parts of the GI tract and the limited absorption of the prodrug there. As it is the sulphapyridine by-product that seems to be responsible for most of the side effects of sulphasalazine (including hypersensitivity reactions), various new azo-bond prodrugs have been developed, substituting sulphapyridine for non-toxic molecules. Dipentum® (olsalazine) comprises an azo-linked dimer of 5-ASA molecules, which is cleaved in the colon to release two molecules of 5-ASA for topical action. Colazal® (balsalazide), a prodrug of 5-ASA attached via an azo bond to 4-aminobenzoyl-β-alanine, releases 99% of the 5-ASA in the colon.
Naturally-occurring polysaccharides which are susceptible to colon-specific microfloral degradation are also being investigated, including amylose, chitosan, guar gum and pectin. The Colal-Pred® delivery system comprises small, prednisolone-containing pellets, coated with a combination of amylase (for colon-specific targeting) and the water insoluble polymer EC (to minimize over-swelling of the highly water-soluble amylase, thereby avoiding premature prednisolone release).
7.6 IMPROVING ORAL BIOAVAILABILITY
7.6.1 FORMULATION STRATEGIES TO IMPROVE ORAL BIOAVAILABILITY
The use of formulations strategies has been investigated in particular for the oral delivery of therapeutic peptides and proteins, such as insulin. The subject has been extensively reviewed (Maher and Brayden 2011; Maher et al. 2014; Park et al. 2011); here, only the most clinically advanced approaches are described.
Low concentrations of surfactants (including sodium lauryl sulfate, polyoxyethylene-9-lauryl ether, and polyoxyethylene-20-cetyl ether) have been studied as absorption enhancers (AEs). Being amphipathic molecules, they can associate with the amphipathic bilayers of the plasma membranes of epithelial cells, thereby enhancing the fluidity and permeability of the bilayer and promoting transcellular transport. Bile acid salts (e.g., sodium taurocholate), and their derivatives, are also thought to function in part via membrane-solubilizing effects.
Other AEs work by transiently loosening the tight junctions (TJs) between cells, thereby facilitating paracellular transport. Many candidates are under study, with medium chain fatty acids such as sodium caprate (a C10 fatty acid) and sodium caprylate (a C8 fatty acid) showing particular promise. At low concentrations, sodium caprate causes a contraction of scaffolding proteins that maintain TJ integrity; this action causes the removal of claudin-5 and tricellulin, thus enhancing paracellular permeability. At higher concentrations, however, its surfactant properties results in mild membrane perturbation, and this may be the initiating event that causes intracellular enzyme cascades that impact the tight junction especially. Sodium caprate is a formulation excipient in GIPET™ (Gastrointestinal Permeation Enhancement Technology), which also uses other AEs, including other medium chain fatty acid derivatives, as well as microemulsion systems based on medium chain fatty acid glycerides. The drug and different AEs are formulated as enteric-coated tablets or capsules. Sodium caprate has also been studied in an enteric-coated oral formulation of antisense oligonucleotides in the 1990s.
Sodium caprylate also enhances absorption via tight junction disruption/paracellular pathway. In the transient permeability enhancer™ (TPE™) technology, the drug is initially solubilized with sodium caprylate and PVP in water. The resulting solution is then lyophilized and the powder is dispersed in a lipophilic medium. This drug/oil suspension is filled into enteric-coated capsules. Trials are ongoing using the TPE™ technology for the oral delivery of octreotide acetate, which is currently given via long-acting injection.
In the Peptelligence™ approach, a number of complementary factors combine to enhance absorption for peptides. An enteric coating on the tablet provides initial protection in the acidic stomach. In the duodenum, the enteric coating dissolves and a disintegrant in the formulation ensures the tablet contents are rapidly released. Citric acid, contained within the core, quickly reduces the intestinal pH in the immediate vicinity. Pancreatic proteases, which have a neutral/alkaline pH range for activity, are thus prevented from degrading the API in this acidic microenvironment. The simultaneous release of lauroyl-L-carnitine, a C12 fatty acid acylcarnitine, further enhances peptide absorption, again by transiently loosening the tight junctions and increasing paracellular transport. The technology is used in Oracal®, an oral formulation of calcitonin for the treatment of osteoporosis, which has recently completed a Phase 3 clinical trial.
A protein oral delivery (POD™) technology for the oral delivery of peptides and proteins uses EDTA as an AE, in combination with a protease inhibitor (e.g., soybean trypsin inhibitor) and a lipoidal carrier (vegetable or fish oil or a synthetic omega-3 fatty acid), administered as enteric-coated capsules. The chelating agent EDTA forms complexes with calcium ions and thus ruptures tight junctions, again facilitating absorption via the paracellular pathway. Listed in the USP, EDTA is considered nontoxic and nonirritant at “allowable” levels.
To be successful, the membrane-perturbing effects of AEs must be local, rapid, and mild—otherwise, there could be a risk of more permanent membrane damage, especially for drugs requiring repeat administration. Given the possible safety risks, as well as the cost of working with new chemical entities as AEs, the pharmaceutical industry has focused on simple enhancers that have a history of safe use and are designated generally regarded as safe (GRAS) status based on prior use as food additives, or FDA “allowed” excipients that can be manufactured cheaply (Maher et al. 2009). Multiple clinical trials of a range of enhancers suggest that the most advanced candidates have good safety profiles. It should also be remembered that the GI tract is sufficiently robust to withstand the daily onslaught and damage afforded by food and fluid intake, as well as bile and gastric secretions. The robustness of the GI tract can be attributed to a number of factors, including the rapid and efficient repair of the GI epithelium within hours, rapid turnover of the epithelial lining in 4–5 days, and dilution effects in the aqueous GI contents. A further issue regarding the safety of AEs is the risk of possible bystander absorption, whereby breaching the membrane barrier could inadvertently facilitate the entry of potentially harmful agents such as bacteria and viruses. This risk is unproven to date, but seems unlikely, given that the extent of tight junction opening and the induced membrane porosity is orders of magnitude smaller than the size of bacteria and viruses.
Research into the use of EIs has particularly focused on the use of proteolytic EIs, in order to enhance the oral absorption of peptide and protein drugs (Bernkop-Schnürch 1998). A wide variety of peptidase inhibitors (PIs) have been studied in man, including soybean trypsin inhibitor and aprotinin, although both have been associated with pharmacology and safety issues. Some recently identified EIs include chicken and duck ovomucoids, derived from the egg white of avian species, which show promise in the protection of insulin from pancreatic enzymes, but do not have associated safety problems.
There is, however, a lack of specificity when using EIs: an incorporated EI may inhibit the breakdown of proteins from dietary sources, thereby decreasing their absorption, with possible toxic consequences. Efforts have been directed toward improving EI specificity. Examples include the Peptelligence™ technology, outlined in Section 7.6.1.1, whereby a localized release of citric acid confines the inhibition of pancreatic enzyme activity to the immediate vicinity surrounding the tablet. Similarly, the protease inhibitor used in the POD™ technology (also outlined in Section 7.6.1.1) is contained within a lipoidal carrier, again to enhance specificity.
A further approach to protect labile molecules involves the physical complexation of an API with an absorption-promoting carrier molecule. Nα-deoxycholyl-L-lysyl-methylester (DCK), a dihydroxyl bile acid derivative, is synthesized by conjugating the amino acid, L-lysine, with deoxycholate. Being positively charged, DCK can physically form a complex in solution with the anionic residues of a drug molecule. The reversible association of DCK with insulin offers protection to insulin from intestinal protease degradation. Absorption may also be enhanced due to the increased lipophilicity of the complex and (given that the carrier is a bile acid derivative) the possibility of enhanced interaction with the bile acid uptake transporters present in the GI tract.
As described in Chapter 3, the solubility of an API is a crucially important factor for oral bioavailability. A drug must initially dissolve in the GI lumen contents, prior to permeating the epithelial layer. Poorly soluble drugs will thus demonstrate limited oral absorption. Furthermore, poor solubility results in a low concentration gradient across the enterocytes, which is a poor driver for transepithelial transport. Poorly water-soluble drugs also demonstrate a lack of dose proportionality, as well as significant intra- and intersubject variability, which is exacerbated by the presence, or absence, of food and fluid in the GI lumen. A variety of formulation approaches are used to improve the solubility of poorly water-soluble drugs and these are discussed fully in Chapter 3.
7.6.1.4 Mucoadhesives and Mucolytics
Widely studied mucoadhesives include Carbopol® (carboxypolymethylene), a synthetic copolymer of acrylic acid and allyl sucrose, which has shown enhancing effects for oral insulin in rodents. Chitosan, a cationic polysaccharide comprising copolymers of glucosamine and N-acetylglucosamine, is strongly mucoadhesive, due to the formation of hydrogen and ionic bonds between the positively charged amino groups of chitosan and the negatively charged sialic acid residues of mucin glycoproteins. This polymer also has some absorption-enhancing effects, as it causes opening of tight junctions, which facilitates the paracellular transport of hydrophilic compounds. Thiolated polymers (thiomers) are mucoadhesive polymers with thiol-bearing side chains, capable of not only physical interaction with mucus but also actual covalent bond formation between the thiol groups of the polymers and cysteine-rich subdomains of mucus glycoproteins. These polymers also have permeation enhancement action and can inhibit P-gp efflux pumps. Preclinical studies have shown the potential of thiomers for oral insulin enhancement. ThioMatrix is exploiting this potential for the noninvasive delivery of a variety of APIs, using their proprietary Thiomer Technology™.
There is again, however, a lack of specificity with this formulation approach: mucoadhesion cannot be targeted to a specific area of the SI, but may occur much higher in the GI tract, for example, in the stomach, where the mucus lining is much thicker than in the SI. A further limitation is the rapid turnover of intestinal mucus, which will limit the amount of time the drug can be realistically retained at the absorbing surface. A mucoadhesive system may be sloughed off and released into the lumen, without ever gaining access to the enterocyte surface (Ensign et al. 2012).
A different line of research is focused on the use of mucopenetrating polymers and mucolytics, in order to minimize the mucus diffusional barrier and thus improve access to the apical membrane of the enterocytes. Mucolytics, such as acetylcysteine, can minimize the diffusional barrier of the overlying mucus gel layer of the GI epithelium. Hydrophilic PEG, hydroxypropyl methacrylate (HPMA), and poly(sialic acid) confer a “slippery surface” on nanoparticles, so that entrapment in the mucus glycoprotein meshes can be avoided. However, this hydrophilic surface coating may subsequently limit uptake via passive diffusion through the hydrophobic cell membranes of the enterocytes.
7.6.2 MICRO– AND NANOPARTICULATE DRUG DELIVERY SYSTEMS
From the early 1980s, a wide array of micro- and nanoparticulate DDS have been intensively investigated as carriers to facilitate oral drug delivery and targeting; some excellent reviews are available (Pouton and Porter 2008; Bakhru et al. 2013; Yun et al. 2013). Studies have focused in particular on the oral delivery of peptides and proteins, especially insulin; the current state of research spans from proof-of-concept studies to late-stage clinical studies.
Nanocarriers investigated for the oral route include microparticles, microspheres, nanoparticles, nanocapsules, liposomes, polymeric micelles, niosomes, dendrimers, SLNs, and microemulsions. A wide range of polymers, including physiological lipids, as well as synthetic, semisynthetic, and natural polymers (including poly(alkyl cyanoacrylates), PLGA, chitosan, alginate, and poly(ethylenimine)), have been studied for the fabrication of nanocarriers. Micro- and nanoparticulate DDS can enhance oral bioavailability via a number of different mechanisms, as outlined in Box 7.1.
Targeting ligands can be associated with nanocarriers, to facilitate cell-specific uptake (Box 7.1). Coupling the targeting vector to a nanoparticulate carrier, rather than an API per se, has the advantage that the conjugation in no way interferes with the biological activity of the API; it also allows for high drug loading. Many types of ligands for epithelial targeting have been described, including lectins, sugars, vitamins (in particular, vitamin B12), peptides, cholesterol, folic acid, and albumin (Li et al. 2014). However, it should be remembered that targeting vectors are only beneficial if the delivery system can actually gain proximity to the corresponding cellular target, but this is in no way guaranteed. Many barriers (including the GI luminal contents, the mucus layer, the presence of enzymes and bile salts) can prevent access of the DDS to the enterocyte surface. Other problems with the approach include low, or variable, receptor expression; possible carrier saturation; and interference with dietary absorption mechanisms.
BOX 7.1 MECHANISMS BY WHICH DRUG DELIVERY SYSTEMS CAN ENHANCE ORAL BIOAVAILABILITY
• Improved drug solubilization
• Protection from enzymatic degradation
• Enhanced interfacial area
• Increased lipidity
• Enhanced transepithelial transport
• Possibility of enhanced lymphatic uptake
• The capacity to incorporate:
• Targeting ligands
• Mucoadhesives/mucolytics
• Enzyme inhibitors
• Absorption enhancers
• Solubility enhancers
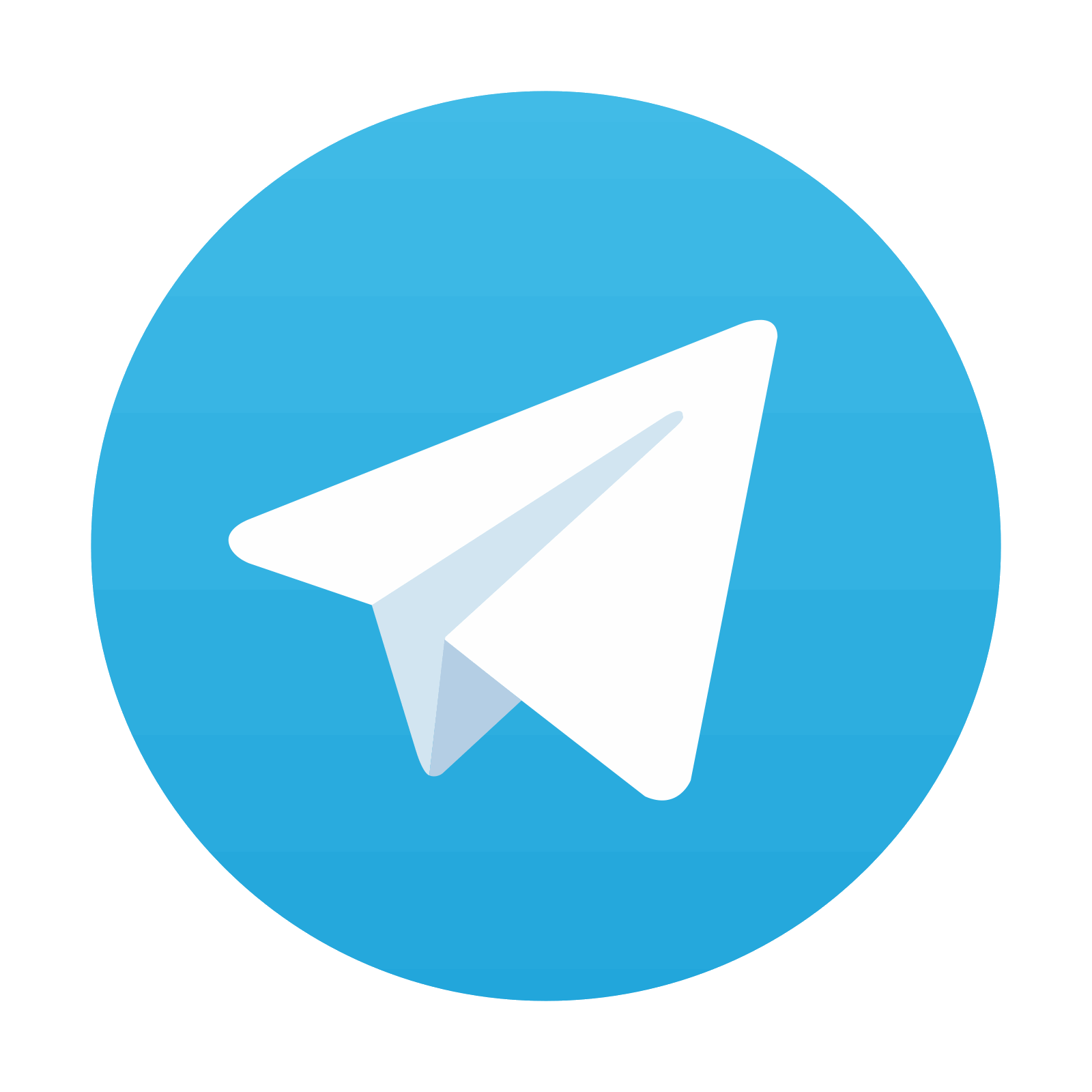
Stay updated, free articles. Join our Telegram channel
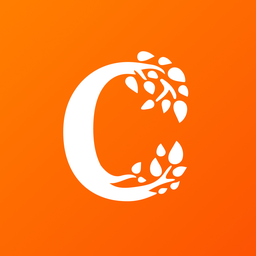
Full access? Get Clinical Tree
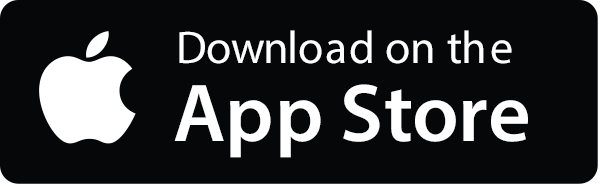
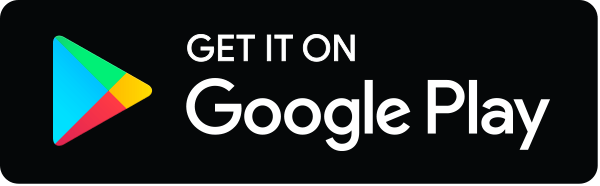