Figure 4-1. Interactions between environment and genetics dictate phenotype. A: Environment alters genetics over millennia, while the effects of environment on epigenetics take place over generations. In the case of humans, genetics dictates our behavior which in turn has led us to create an obesogenic environment, creating a vicious cycle that exacerbates the obesity epidemic. B: The obesity phenotype blossoms in a modern obesogenic environment, with the population body mass index (BMI) curve broadening and shifting rightward, with an increase in median BMI. The increase in median BMI is relatively modest, but a substantially larger percentage of people populate overweight and obese BMI ranges. Of note, the schematic graphs shown are similar to those that describe the shift in US population BMI National Health and Nutrition Examination Survey data from the late 1970s to the early 2000s.
PHYSIOLOGY OF OBESITY
3 The adipostat: A dominant determinant of systemic metabolic homeostasis is the quantity of existent adipose tissue stores, the adequacy of which is determined by the hypothalamic feeding center (HFC). The HFC receives multiple afferent neural and hormonal inputs from all organ systems, including adipose tissue, and orchestrates a range of responses via efferent outputs that regulate all aspects of metabolism. These multiple HFC-regulated systems monitor and maintain adipose tissue stores, and together comprise the adipostat, the sum total of all processes that defend body weight. The amount of adipose tissue deemed adequate by the HFC varies among individuals and is dictated by multiple poorly understood thrifty genes. Collectively, these genes and the proteins they regulate define the adipostat set-point, which determines the degree of metabolic thrift of an individual. The hypothalamus of a lean person “considers” a lower amount of adipose tissue to be adequate, while the hypothalamus of an obese person requires higher levels of baseline adipose tissue stores. Weight loss alerts the HFC to deviation from the adipostat set-point and triggers robust compensatory metabolic responses that act to restore adipose tissue mass to baseline levels, including but not limited to increased hunger, decreased satiety, and decreased metabolic rate. The HFC resides deep in the midbrain, far removed from the frontal cortex, the seat of conscious thought and what we refer to as “willpower.” HFC-mediated metabolic responses are characterized by tight regulation; deviation outside the range of these responses for more than limited periods of time is not possible based on conscious choice, explaining the near-universal failure of conscious dietary efforts to lose weight. Obese patients are able to control their weight only to a point, and only for brief periods, after which compensatory responses are activated. These mechanisms also explain the lack of efficacy of liposuction as a tool for weight loss. Regardless of the method, whether diet-induced or liposuction, reduction in adipose tissue mass activates compensatory mechanisms that act to restore adipose tissue mass to set-point levels. Bariatric surgery-induced weight loss is the only exception to this rule, inducing poorly understood paradoxical responses that bypass the adipostat, including decreased satiety and increased metabolic rate.
Multiple physiologic mechanisms comprise the adipostat and regulate body weight. Most important in humans are the collective satiety and hunger systems that regulate food intake. Less powerful but nonetheless important are mechanisms that control metabolic rate, adipocyte physiology, and the microbiome.
4 Satiety and hunger: Regulation of food intake is the dominant mechanism by which humans control body weight. This is not true for all species. While food intake is an important mechanism of energy homeostasis in mice, regulation of metabolic rate plays a greater role in mice than in humans. Food intake in humans is controlled by a family of proteins that regulate satiety and hunger primarily by acting on receptors within the hypothalamus. These diverse proteins are secreted by multiple organ systems, including but not limited to adipose tissue, the gut, the liver, and the skeletal muscle.
The Ob mouse, a genetic mutant strain described in 1950, manifests an obese phenotype, along with a range of other abnormalities related to immune, endocrine, and reproductive functions. The Ob mouse harbors an inactivating point mutation in the leptin gene (Gr. leptos, thin). Leptin is a 16-kD satiety hormone secreted by adipose tissue in response to a meal that in turn acts on receptors within the HFC to induce satiety. Leptin establishes communication between adipose tissue, the gut, and the brain that signals the status of adipose tissue stores to the brain, which in turn dictates food intake. As such, leptin represents a paradigmatic mediator of the adipostat, and an example of the complex interorgan communication that underlies its function (Fig. 4-2). Soon after the cloning of the leptin gene in 1994,9 rare humans with leptin mutations were identified with an Ob phenotype that was reversed with administration of exogenous recombinant leptin. Unfortunately, similar therapy was ineffective in common human obesity, which is not due to a single-leptin mutation, but rather is polygenic and characterized by hypothalamic resistance to leptin satiety effects. Nonetheless, the discovery of leptin led to an explosion of satiety and hunger research, and over the ensuing decade multiple satiety and hunger proteins were described.
Figure 4-2. Leptin mediates communication between adipose tissue, gut, and brain. A: Signals from the gastrointestinal tract triggered by a meal circulate to adipose tissue and induce adipocyte leptin secretion. Leptin circulates to the hypothalamus, where it binds receptors in the arcuate nucleus to induce satiety, leading to decreased food intake. Leptin is one of many signals that mediates bidirectional communication between gut, adipose tissue, and brain. Other organ systems are also involved, including immune and reproductive systems and the liver. B: Leptin regulates allostatic control of short-term and long-term food intake. Postprandial leptin secretion induces satiety for a period of hours, after which leptin levels and satiety wane, prompting food intake. Similarly, leptin, along with other mediators of food intake, controls long-term weight regulation: diet-induced weight loss leads to decreased adipose tissue mass, reducing peak postprandial leptin levels, leading to decreased postprandial satiety and increased food intake at each subsequent meal until adipose tissue mass is restored to set-point levels. In this manner, leptin functions as a component of the adipostat. This allostatic control provides an explanation for rebound weight gain after diet-induced weight loss.
The control of feeding behavior and nutrient intake is a highly regulated process centered in the hypothalamus, which integrates information regarding nutritional status, environment, and energy expenditure via central and peripheral orexigenic and anorexigenic signals. Peripheral messengers include signals from adipose tissue (adipokines), including leptin and adiponectin, cytokines, such as TNF-α and interleukin (IL-6), and gut peptides. Among this diverse latter class of mediators, ghrelin is a dominant stimulant of feeding. Ghrelin is expressed primarily by oxyntic glands in the fundus of the stomach. Gastric ghrelin–producing cells represent about one-fourth of endocrine cells within the gastric mucosa. Ghrelin is also produced in the hypothalamus.
Encoded by five exons, preproghrelin undergoes endoproteolytic processing and posttranslational modification to yield des-acyl ghrelin and acyl ghrelin. Both hormones share the same amino acid sequence, and both are detectable in blood, but acyl ghrelin, which undergoes acylation of the Ser3 residue, is the active form. Acyl ghrelin regulates feeding, metabolic activity, and insulin secretion. The enzyme-mediating acylation is the membrane-bound ghrelin O-acyltransferase (GOAT). Genetic disruption of the GOAT gene in mice leads to complete absence of acyl ghrelin. GOAT inhibition improves glucose tolerance and reduces weight gain in mice. Plasma acyl-ghrelin levels increase with fasting and decrease after feeding, a pattern indicating that ghrelin is involved in meal initiation. The ghrelin receptor is a member of the family of G protein–coupled receptors and contains seven transmembrane domains. Ghrelin receptors are widely distributed among both central and peripheral tissues, including the pituitary gland, hypothalamus, pancreas, stomach, and intestine. Ghrelin causes growth hormone secretion following peripheral or central administration, and release of growth hormone from cultured pituitary cells.
Ghrelin is the only orexigenic hormone identified to date. The relative scarcity of orexigenic proteins relative to anorexigenic proteins underscores an important fundamental characteristic of the global regulation of food intake, which is biased toward chronic basal hunger regulated by multiple satiety factors (rather than chronic basal satiety regulated by multiple hunger factors). This central feature of food intake control systems predisposes to excess food intake and thus provides a selective advantage in environments of food scarcity during our evolution, but leads to obesity in our modern environment. In humans, the intravenous administration of ghrelin at physiologic concentrations induces the sensation of hunger and stimulates oral intake. Circulating ghrelin levels peak just prior to meal initiation and decline rapidly postprandially. Ghrelin secretion is increased by weight loss and by restriction of caloric intake. Serum ghrelin levels are increased in anorexic individuals and depressed in obese subjects. In animals, ghrelin administration has been found to stimulate food intake, to induce growth of adipose tissues, and to increase body weight. The administration of ghrelin antibody or ghrelin receptor antagonists blunts ghrelin-induced weight gain and positive energy balance. Similar molecules are under active study as potential therapeutic agents.
Ghrelin is a circulating hormone with CNS effects. The arcuate nucleus of the hypothalamus is a crucial site for the integration of fasting and feeding signals. Two types of neurons, with opposing actions on feeding behavior, have been identified in the arcuate nucleus. Neurons that express proopiomelanocortin and cocaine- and amphetamine-regulated transcript (CART) suppress food intake, reduce body weight, and increase energy expenditure. In contrast, neurons producing neuropeptide Y and agouti gene–related transcript (AgRP) are orexigenic. These cells act to stimulate food intake and reduce energy expenditure. Ghrelin, as well as leptin and multiple other gut peptides, adipokines, and cytokines, directly mediate the activities of these two types of neurons (Fig. 4-3). Direct peripheral effects of ghrelin on peripheral tissues also contribute to the regulation of body weight and energy homeostasis.
Leptin and ghrelin are paradigmatic adipokine and gut peptide mediators of food intake respectively, but multiple other proteins contribute. Adipokines that regulate satiety include adiponectin, visfatin, apelin, and lipocalin; gut peptides include glucose-dependent insulinotropic peptide (GIP), glucagon-like peptide-1 (GLP-1), nesfatin-1, oxyntomodulin, pancreatic polypeptide, cholecystokinin, amylin, glucagon, somatostatin, cholecystokinin, and insulin. Finally, cytokines, including TNF-α and IL-6, in addition to immunoregulatory functions, also play important roles in the control of food intake. TNF-α, for example, mediates anorexigenic responses in the context of cachexia and inflammatory states. Virtually all adipokines, gut hormones, and cytokines have multiple overlapping functions that include regulation of satiety and hunger, immune function, glucose homeostasis, lipid metabolism, and endocrine and reproductive function (Fig. 4-4). This functional diversity speaks to the intimate association of energy homeostasis with all aspects of physiology.
Genetic polymorphisms in the melanocortin 4 receptor gene, which regulates satiety and hunger responses and the HFC set-point, are implicated in 5% of cases of human obesity. Similar polymorphisms associated with obesity exist with the genes encoding leptin and its receptor, ghrelin, neuropeptide Y, adiponectin, GLP-1, and other genes associated with the control of satiety and hunger. These observations demonstrate that variability in the genes that regulate food intake, with corresponding variability in the functional control of satiety and hunger, contribute to the development of human obesity.
Metabolic rate: While less important than control of food intake, variability in metabolic rate contributes to the pathogenesis of obesity. In obese subjects who lose weight with diet, total energy expenditure is decreased up to 20% beyond that expected by loss of fat and fat-free mass alone, and is accompanied by a corresponding decrease in voluntary physical activity.10 In contrast to diet-induced weight loss, bariatric surgery-induced weight loss paradoxically induces increased energy expenditure, which may explain the efficacy of surgery. In the absence of surgery however, compensatory decreases in energy expenditure counteract caloric restriction and contribute to adipostat responses that resist declines in adipose tissue mass. Studies of overfeeding and weight gain in obese subjects demonstrate converse changes with a compensatory increase in total energy expenditure. Importantly, overfeeding studies in twin cohorts demonstrate a significant genetic component to variability in energy expenditure responses to overfeeding, suggesting that variability in metabolic rate responses to weight loss contributes to the development of obesity in those at risk.3
Differences in sympathetic nervous system activity are observed in humans and represent a potential mechanism underlying variability in metabolic rate. Obese humans demonstrate decreased sympathetic nervous system activity compared to lean humans, and higher levels of sympathetic nervous system activity predict successful diet-induced weight loss. Differences in endocrine responses also contribute, with obese humans manifesting differences in thyroid hormone and catecholamine balance associated with decreased metabolic rates. At the cellular level, differences in energy utilization and thermogenesis play an important role. Skeletal muscle energy utilization efficiency is increased in humans who achieve successful weight loss and decreased in those who gain weight. Furthermore, decreased levels of skeletal muscle nonexercise-induced thermogenesis and diet-induced thermogenesis have been demonstrated in obese humans.11 These observations suggest that fundamental differences in cellular energy homeostasis contribute to obesity. The mechanisms underlying these variable cellular responses are not fully defined, but differences in uncoupling protein (UCP) function are implicated. UCPs uncouple oxidative phosphorylation from electron transport in mitochondria, creating a proton leak that generates heat rather than ATP. The role of UCPs in the pathogenesis of obesity is an important area of active research. UCP function is highly variable and genetically determined in mice, and polymorphisms in UCP genes correlate with obesity and metabolic disease in humans, supporting a role for genetic variability in UCP function in obesity pathogenesis. Transgenic mice overexpressing UCP-1 under control of the adipose tissue-specific AP2 promoter are resistant to obesity, suggesting the potential therapy for obesity based on manipulation of cellular thermogenesis and metabolic rate.
Figure 4-3. A simplified schematic of the hypothalamic feeding center and its primary afferent signals. A. Multiple hormones secreted by the gastrointestinal tract and adipose tissue act as afferent signals that impact on the arcuate nucleus (ArcN) within the hypothalamic feeding center to regulate feeding behavior. B. Peripheral afferent signals impact on first-order ArcN neurons, which in turn communicate with second-order paraventricular nucleus (PVN) and lateral hypothalamic area (LHA) neurons to coordinate behavioral and metabolic output. PVN signaling is primarily anorexigenic and catabolic, and is enhanced by leptin and insulin, while LHA signaling is primarily orexigenic and anabolic and inhibited by leptin and insulin. Higher-order anorexigenic PVN outputs include corticotropin-releasing hormone (CRH), oxytocin, and thyrotropin-releasing hormone (TRH); higher-order orexigenic LHA outputs include melanin-concentrating hormone (MCH) and orexins A and B. Both pathways negatively regulate the other. Other peripheral and central mediators stimulate first- and second-order neurons as well, including ghrelin, CCK, GLP-1, serotonin, endogenous cannabinoids, and norepinephrine.
Adipocyte physiology: Adipocytes arise from mesenchyme-derived adipocyte stem cells within adipose tissue. Nomenclature is evolving and includes the terms preadipocyte or adipocyte/adipose tissue stem cell. Adipocyte stem cells are pluripotent, and depending on the stage of differentiation, may give rise to adipocytes, fibroblast, myocytes, and other cell types. Multiple adipocyte stem cell subpopulations give rise to adipocytes of variable phenotypes, including white, brown, and beige. Evidence suggests that hematopoietic precursor cells may also give rise to adipocytes.
Until recently it was thought that adipocyte stem cell proliferation, that is, hyperplasia, did not occur in postnatal humans, and that adipocyte hypertrophy was the primary mechanism of increased adipose tissue mass in obesity. Recent data suggest that both hyperplasia and hypertrophy contribute to evolving obesity, with hyperplasia predominating during childhood and hypertrophy predominating during adulthood. These data derive in part from ingenious methods studying incorporation of C14 into adipocyte DNA in individuals born before and after mid-20th century nuclear bomb testing, which raised environmental C14 levels.12 Data over the last decade have demonstrated that adipocyte hyperplasia and proliferation are highly regulated and that aberrations in these processes contribute to obesity. Adipocyte necrosis and apoptosis as a result of hypoxia and nutrient excess (described below) are matched by increased adipocyte stem cell proliferation and differentiation. Furthermore, adipocytes from obese humans demonstrate increased hyperplastic and hypertrophic capacities, contributing to increased adipose tissue mass with progressive obesity.13 The epigenetic programming of adipocyte stem cells during fetal development may underlie some of these differences in adipocyte behavior observed between obese and lean subjects. Defining regulatory mechanisms of adipocyte growth is an active area of research and will lead to methods to manipulate adipocyte biology and treat obesity at its source.
Figure 4-4. Primary peptide mediators of energy homeostasis. Cytokines, adipokines, and gut peptides regulate diverse aspects of metabolism to control global energy homeostasis. Each of these proteins has dominant functions within its primary family, but also demonstrates cross-regulatory functions in other domains. In addition, mediators participate in crosstalk at the level of cellular receptors and downstream intracellular signaling mediators. Shown are only partial lists of peptides and the physiologic functions they control.
The microbiome: In a very real sense, the microbiome may be considered a distinct organ system much like cardiopulmonary, gastrointestinal, or renal systems, and like any organ system, aberrations in the microbiome contribute to the pathophysiology of disease. The microbiome plays an important role in energy homeostasis. Animals raised in germ-free environments manifest increased food intake but decreased body weight and do not develop obesity and insulin resistance when fed a high-fat diet, demonstrating that gut microbiota contribute to nutrient digestion. Gut microbiota ferment nonhost-digestible polysaccharides into absorbable monosaccharides and short-chain fatty acids that are absorbed by host enterocytes and colonocytes. Products of microbiota fermentation act not only as nutrients, but also regulate host metabolism: short-chain fatty acids induce host satiety, mediated in part by increased secretion of GLP-1 and peptide YY and decreased secretion of ghrelin. Separate data demonstrate interactions between microbiota and adipose tissue, liver, skeletal muscle, and CNS. These effects are mediated by short-chain fatty acids, activation of Toll-like receptors on host cells via lipopolysaccharide and other bacterial products, regulation of host systemic and cellular metabolism, and other diverse mechanisms, all of which affect diverse aspects of host energy homeostasis.
Alterations in microbiome–host interactions contribute to the pathogenesis of obesity and metabolic disease. Germ-free mice, when colonized with stool from obese mice, gain more weight than mice colonized with stool from lean mice, demonstrating an obesity-specific microbiome. The ratio of gut Firmicutes/Bacteroidetes species is increased in obese mice and humans. Ongoing research has begun to identify specific subspecies within these broad categories of bacteria that are altered in obesity and metabolic disease to provide a higher-resolution picture of the microbiome in obesity. For example, obesity in mice and humans is associated with an increase in gram-negative gut bacteria, with a concomitant increase in lipopolysaccharide absorption from the gut, which has been postulated to contribute to obesity-associated inflammation and insulin resistance. The tools used to evaluate the microbiome are rapidly evolving. Sequencing of the microbial genome using ribosomal 16S is well established, while evolving technologies include shotgun sequencing of the entire metagenome and functional assays involving transfer of human microbiota in the form of stool into germ-free animals to study in vivo effects. Manipulation of the microbiome holds significant promise. Prebiotic (nondigestible bacterial nutrients) and probiotic (specific bacterial subspecies thought to confer systemic benefits) therapies are areas of active research, while antibiotic therapy directed against gram-negative bacteria reduces steatosis and systemic inflammation in obese rodents.
Summary: Satiety and hunger, metabolic rate, adipocyte physiology, and the microbiome are but a few of the many processes that regulate metabolism and are dysregulated in obesity. Differences in lipid and glucose metabolism, immune function, central and peripheral nervous system function, and multiple other processes that impact upon energy metabolism contribute to the wide variability of the obesity phenotype in humans. This variability is the result of genetic polymorphisms that contribute to metabolic diversity among humans and the wide range in human body habitus. The question is often posed as to whether obesity should be considered a disease. Rather, obesity is better thought of as a maladaptive compensatory response to an environment that is radically different than that in which our species evolved. The obesity phenotype remained relatively underexpressed in environments of food scarcity in which we evolved, in which obesogenic genes acted to defend adipose tissue stores and enhance survival, but blossoms in our modern environment. An understanding of this intimate interplay between phenotype and environment will lead to novel therapy for obesity based on manipulation of environment and genetics.
PATHOPHYSIOLOGY OF METABOLIC DISEASE
Obesity and metabolic disease: Obesity is associated with a range of pathology involving every organ system collectively referred to as metabolic disease. While underlying mechanisms are multiple, the root cause of all metabolic disease is a failure of adipose tissue nutrient buffering capacity, leading to overflow of nutrients, metabolites, cytokines, and adipokines from adipose tissue to other tissues. The clinical effects of overflow differ depending on the target tissue, but the fundamental cellular responses to nutrient excess common to all tissues, adipose included, involve mechanisms by which cells control nutrient flux and respond to nutrient excess. These cellular responses underlie the pathophysiology of metabolic disease.
5 Metabolism, nutrient excess, and the root cause of metabolic disease: Energy homeostasis is the dominant and overriding goal of all biologic systems. Metabolism (Gr. metabole, change) may be defined as the sum total of all chemical reactions and physiologic processes that regulate cellular and systemic energy homeostasis. At the cellular level, metabolic processes are fundamental to all metazoan life and are highly conserved across phyla; examples include the citric acid cycle and mitochondrial respiration, which utilize multiple enzymes, the structure and function of which are conserved from yeast to humans. At the systemic level, metabolic processes are central to maintaining homeostasis and interface with virtually with every organ system and every physiologic process. The basic substrates of metabolism are the macro- and micronutrients that comprise the stuff of cells and tissues, the fatty acids, amino acids, and carbohydrates and their derivatives that are the constituents of complex macromolecules, and the micronutrients that contribute to the catalysis of reactions that synthesize and degrade macronutrients and higher-order macromolecules. In one sense, all cells are simply clearinghouses for nutrients. Cellular nutrient flux provides substrate for synthesis of macromolecules via anabolic metabolic processes, which are in turn degraded via catabolic metabolic processes. Maintaining the balance between anabolism and catabolism at cellular and systemic levels is of critical importance. Cancer, for example, may be viewed as a metabolic imbalance that favors cellular anabolism over catabolism. Cachexia, in contrast, may be considered as a metabolic disorder that favors systemic catabolism over anabolism.
The nutrient flux with which cells are constantly faced determines the balance between anabolism and catabolism and dictates organism-wide energy homeostasis. Nutrients are, by their very nature, high-energy capacity molecules, much like oxygen, and like oxygen, are capable of participating in energy-intensive and potentially damaging reactions. Fatty acid metabolites such as ceramides and diacylglycerols, and glucose metabolites including advanced glycation end products and glucosamines, trigger inflammation, oxidative stress, and insulin resistance at the cellular level.
Excess nutrients are therefore potentially damaging to cells, and cellular physiology has evolved processes designed to control exposure, flux, and sequestering of these high-energy molecules. These cellular processes were selected by evolution to manage transient nutrient excess, but are not well adapted to manage chronic nutrient excess, a situation that cells only rarely encountered during evolution. Maladaptive cellular responses to chronic nutrient excess underlie the pathogenesis of all obesity-related disease and occur in all cells, explaining the involvement of virtually every organ system in metabolic disease. These processes consist of a triumvirate of fundamental cellular stress responses: endoplasmic reticulum (ER) stress, oxidative stress, and inflammation.
Cellular responses to excess nutrient flux: The ER is a complex organelle present in virtually all eukaryotic cells that orchestrates synthesis, folding, and intracellular transport of macromolecules. The rough ER, studded with ribosomes, is predominantly responsible for protein synthesis, while the smooth ER is predominantly responsible for lipid and carbohydrate synthesis. The ER receives a constant influx of nutrient substrates, which it sequesters and directs toward macromolecule synthesis or degradation, thus carefully metering cellular nutrient exposure. In this capacity, the ER acts as a nutrient sensor, responding to changes in cellular nutrient flux by directing cellular anabolism and catabolism accordingly. The ER also responds to other cell stressors, including hypoxia, toxins, disturbances in electrolyte or micronutrient balance, and a host of other stimuli that alter cell homeostasis. Excess cellular nutrient flux, like other forms of stress, leads to a series of stepwise ER responses that are collectively referred to as the ER stress response.
The initial ER stress response to modest increases in nutrient flux involves increased expression of chaperone proteins involved in macromolecule synthesis to accommodate increased anabolism of excess nutrients. If nutrient excess persists to exceed the capacity of cellular macromolecule synthesis and nutrient sequestration, the ER stress response progresses to activate the unfolded protein response. The unfolded protein response downregulates global protein expression while upregulating expression of chaperone proteins in an adaptive response designed to maintain synthesis of essential cellular proteins. If nutrient excess persists beyond the capacity of the ER to maintain proper protein synthesis, then the unfolded protein response progresses to induce cell apoptosis. The unfolded protein response thus protects cells from modest increases in nutrient flux and induces cell death in the face of extreme nutrient excess.
The ER stress response is not the only cellular response to nutrient excess. Macromolecule synthesis generates reactive oxygen species (e.g., superoxides, oxygen/hydroxyl-free radicals, hydrogen peroxide) which, like nutrients, are highly energetic molecules capable of damaging cells. Cells have evolved multiple mechanisms to sequester reactive oxygen species and manage oxidative stress, including antioxidant enzymes such as superoxide dismutase, glutathione peroxidase, and catalase, scavenging molecules such as ascorbic acid, urate, and divalent ions, and the thioredoxin and glutaredoxin systems. The ER and mitochondria are primary cellular sites of control of oxidative stress. When nutrient flux, macromolecule synthesis, and reactive oxygen species production exceed cellular antioxidant capacity, cells activate diverse oxidative stress responses, which increase expression of antioxidant proteins, sequester reactive oxygen species, and increase mitochondrial uncoupling, which transiently reduces cellular reactive oxygen species production. Oxidative stress responses overlap with ER stress responses, and if oxidative stress persists, the unfolded protein response is activated and leads to apoptosis.
The third arm of the cellular stress response is inflammation. Increased nutrient flux generates an inflammatory response designed to scavenge debris and byproducts from increased cell turnover and macromolecule synthesis that result from ER and oxidative stress responses. Testament to the close relationship between metabolism and inflammation, central inflammatory and metabolic mediators demonstrate marked functional overlap. For example, TNF-α and leptin, in addition to their dominant functions in regulating inflammation and satiety respectively, each plays important reciprocal roles in regulating body weight and inflammation.14,15 TNF-α and leptin are but two examples of many satiety and hunger factors and inflammatory cytokines that manifest dual immunoregulatory and energy homeostasis functions. In addition, molecular byproducts of metabolism trigger inflammation. Free fatty acids are ligands for Toll-like receptors, while advanced glycation end products trigger receptors of advanced glycation end-products pathways which directly activate innate immune effector cells, establishing a direct molecular link between metabolism and inflammation. Obesity is associated with a state of chronic systemic inflammation that plays a central role in the pathogenesis of multiple comorbid diseases. Serum cytokine levels are increased in obese animals and humans, and adipose tissue manifests a pan-leukocyte infiltrate, findings that directly correlate with the magnitude of obesity and severity of metabolic disease.
Increased ER stress, oxidative stress, and inflammation have been demonstrated in obese rodents and humans. An important aspect of cellular stress responses is that each potentiates the others via overlap in fundamental cell signaling pathways including PI3-Akt, MAPK, AMPK, mTOR, JAK-STAT, and NFκB. These signaling pathways are triggered by multiple stimuli that are dysregulated in obesity, including adipokines, cytokines, growth factors, and nutrients and metabolites. Furthermore these signaling pathways participate in robust crosstalk at multiple levels, establishing a vicious cycle that perpetuates cell stress in the face of chronic nutrient excess (Fig. 4-5). These processes are common to all cells and affect all tissues in late-stage obesity, explaining the diverse manifestations of systemic metabolic disease. But where do these processes begin, and what initiates them? To answer this question, we must explore the early events in adipose tissue in evolving overweight and obesity – it is within adipose tissue that metabolic disease originates.
Adipocyte hypertrophy, hypoxia, inflammation, and fibrosis: Adipocytes are exquisitely well designed to manage high levels of nutrients. One of the first responses of adipocytes to chronic elevations in nutrient flux in early overweight and obesity is hypertrophy. Free fatty acids are absorbed by adipocytes through pinocytosis mediated by fatty acid-binding proteins, as well as being synthesized from glucose and glutamine via de novo lipogenesis, and stored in lipid droplets via a highly regulated process mediated by perilipin proteins. Virtually all cells are capable of storing lipid to some degree, but adipocytes have an extremely high capacity for lipid storage and hypertrophy. Adipocytes are one of only a few cell types that can enlarge to diameters greater than 100 microns, the diffusion distance of oxygen. Adipocyte diameter in humans correlates directly with the magnitude of obesity and the severity of metabolic disease; adipocytes in lean subjects are virtually all less than 100 microns in diameter, while those in obese subjects are greater than 100 microns, in some cases exceeding 200 microns.16 Progressive adipocyte hypertrophy establishes a state of cellular hypoxia within adipose tissue that has been verified in obese mice and humans. Decreased adipose tissue capillary density and blood flow in obesity exacerbate hypoxia. This hypoxic state has broad effects on adipocyte metabolism, inducing insulin resistance, ER stress, oxidative stress, and inflammation, and inhibiting lipogenesis. Manipulation of hypoxic responses in adipocytes holds therapeutic promise. For example, targeting hypoxia-inducible factor-1α, a dominant cellular hypoxia-response protein, in murine obesity ameliorates metabolic disease.17
Hypoxia induces adipocyte apoptosis and necrosis which in turn recruits an inflammatory response designed to scavenge dead and dying adipocytes. This inflammatory state is a dominant aspect of adipose tissue dysfunction in obesity, and is directly linked to insulin resistance and dysregulation of lipid metabolism at local (adipose tissue) and systemic levels. Central to adipose tissue inflammation is a marked accumulation of adipose tissue macrophages (ATMs), the number of which directly correlates with the degree of obesity in mice and humans. ATMs are important mediators of metabolic disease, and are altered in phenotype, shifting from a scavenging M2 phenotype to an inflammatory diabetogenic M1 phenotype.18 ATMs are a dominant source of inflammatory cytokines, including TNF-α, IL-6, and IL-1, all of which induce insulin resistance and aberrations in glucose and lipid metabolism in adipocytes via multiple mechanisms. In support of a central role for ATMs in the pathogenesis of metabolic disease, mice transgenically engineered to lack macrophage homing molecules in adipose tissue do not develop obesity-related ATM infiltrates and are protected from diabetes. Preliminary trials of pharmacologic agents that interfere with macrophage homing to adipose tissue in humans show promise as treatment for diabetes. Finally, adipose tissue inflammation in obesity involves more than macrophages, and is associated with a pan-leukocyte infiltrate that includes T-cells, B-cells, NK cells, NKT cells, and eosinophils. Therapy for metabolic disease based on nonmacrophage cellular immune mediators is an area of active research.
Figure 4-5. Highly simplified schematic of major cell signaling pathways that regulate energy homeostasis and cell stress responses. Primary stimuli for all cells include adipokines, insulin, IGF-1, nutrients and metabolites including free fatty acids, and their derivatives such as ceramide (which activate Toll-like receptors, TLR), glucose, fructose, and advanced glycation end products (which activate receptors for advanced glycation end products, RAGE), and cytokines. Primary intracellular signaling nexi include mitogen-activated protein kinases (MAPK), PI3-Akt, JAK/STAT signaling mediators, AMP-activated protein kinase (AMPK), mammalian target of rapamycin (mTOR), and NFκB, all of which are linked to ER stress and oxidative stress responses. These pathways engage in highly redundant crosstalk via cross-reactivity of intracellular signaling mediators and induction of gene transcription, and conspire to integrate multiple stimuli to regulate fundamental aspects of cell metabolism, survival, and death in response to nutrient availability and cell stressors such as hypoxia.
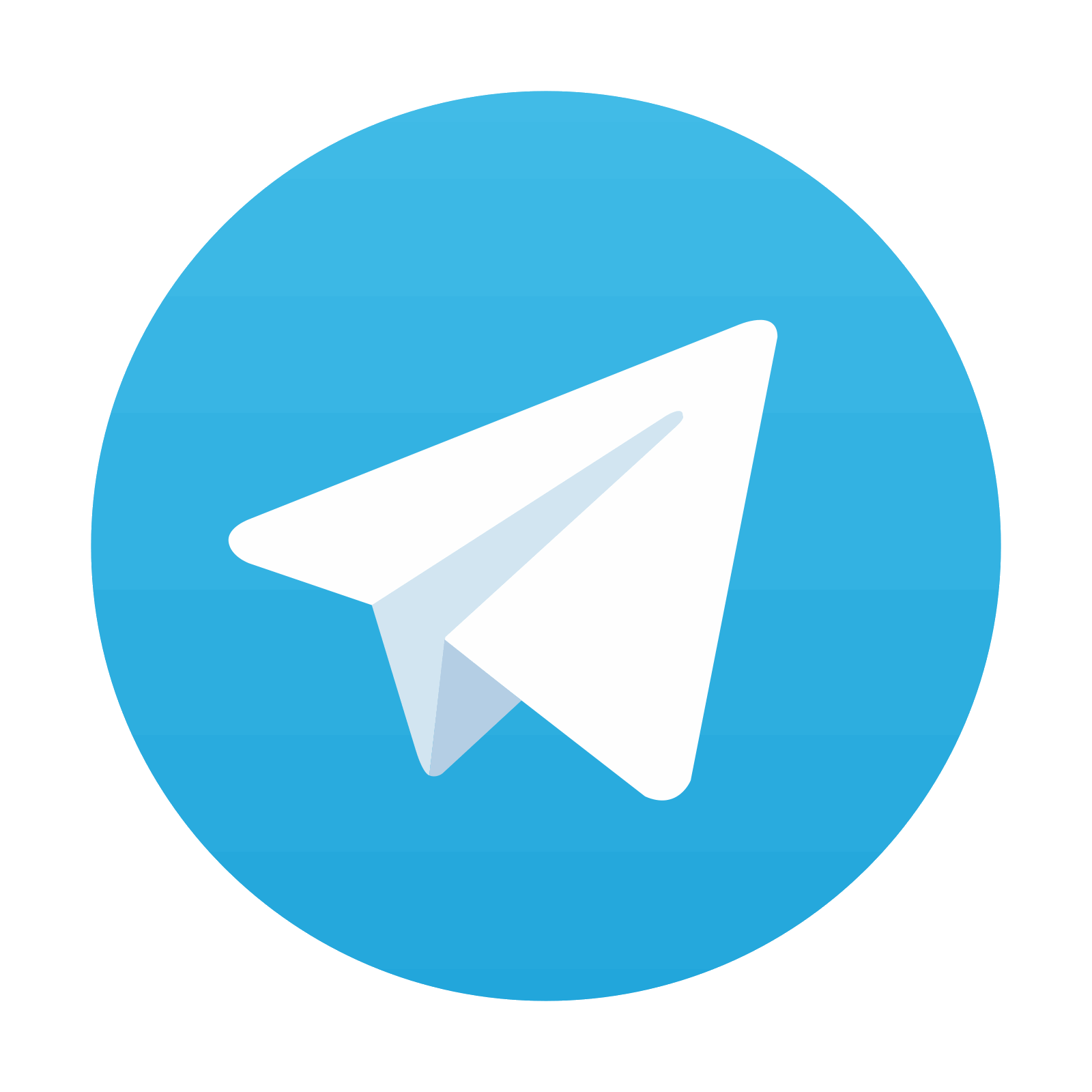
Stay updated, free articles. Join our Telegram channel
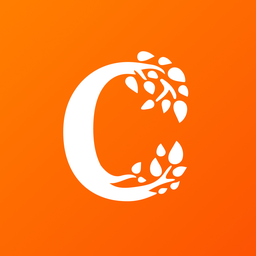
Full access? Get Clinical Tree
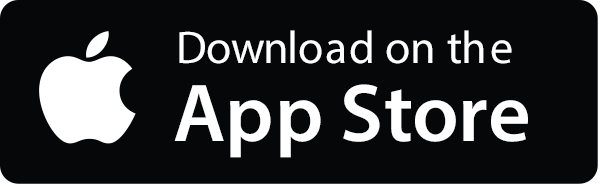
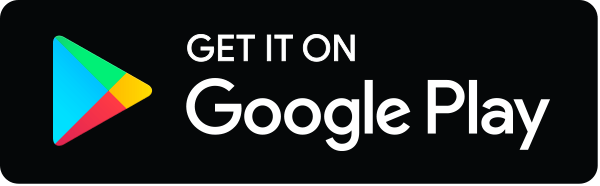