34
Nucleic Acid Structure & Function
OBJECTIVES
After studying this chapter, you should be able to:
Understand the chemical monomeric and polymeric structure of the genetic material, deoxyribonucleic acid, or DNA, which is found within the nucleus of eukaryotic cells.
Explain why genomic nuclear eukaryotic DNA is double stranded and highly negatively charged.
Understand the outline of how the genetic information of DNA can be faithfully duplicated.
Understand how the genetic information of DNA is transcribed, or copied into myriad, distinct forms of ribonucleic acid (RNA).
Appreciate that one form of information-rich RNA, the so-called messenger RNA (mRNA), can be subsequently translated into proteins, the molecules that form the structures, shapes, and ultimately functions of individual cells, tissues, and organs.
BIOMEDICAL IMPORTANCE
The discovery that genetic information is coded along the length of a polymeric molecule composed of only four types of monomeric units was one of the major scientific achievements of the 20th century. This polymeric molecule, deoxyribonucleic acid (DNA), is the chemical basis of heredity and is organized into genes, the fundamental units of genetic information. The basic information pathway—that is, DNA, which directs the synthesis of RNA, which in turn both directs and regulates protein synthesis—has been elucidated. Genes do not function autonomously; their replication and function are controlled by various gene products, often in collaboration with components of various signal transduction pathways. Knowledge of the structure and function of nucleic acids is essential in understanding genetics and many aspects of pathophysiology as well as the genetic basis of disease.
DNA CONTAINS THE GENETIC INFORMATION
The demonstration that DNA contained the genetic information was first made in 1944 in a series of experiments by Avery, MacLeod, and McCarty. They showed that the genetic determination of the character (type) of the capsule of a specific pneumococcus could be transmitted to another of a different capsular type by introducing purified DNA from the former coccus into the latter. These authors referred to the agent (later shown to be DNA) accomplishing the change as “transforming factor.” Subsequently, this type of genetic manipulation has become commonplace. Similar experiments have recently been performed utilizing yeast, cultured plant and mammalian cells, and insect and mammalian embryos as recipients and molecularly cloned DNA as the donor of genetic information.
DNA Contains Four Deoxynucleotides
The chemical nature of the monomeric deoxynucleotide units of DNA—deoxyadenylate, deoxyguanylate, deoxycytidylate, and thymidylate—is described in Chapter 32. These monomeric units of DNA are held in polymeric form by 3’,5’-phosphodiester bonds constituting a single strand, as depicted in Figure 34–1. The informational content of DNA (the genetic code) resides in the sequence in which these monomers—purine and pyrimidine deoxyribonucleotides—are ordered. The polymer as depicted possesses a polarity; one end has a 5′-hydroxyl or phosphate terminal while the other has a 3′-phos-phate or hydroxyl terminal. The importance of this polarity will become evident. Since the genetic information resides in the order of the monomeric units within the polymers, there must exist a mechanism of reproducing or replicating this specific information with a high degree of fidelity. That requirement, together with X-ray diffraction data from the DNA molecule and the observation of Chargaff that in DNA molecules the concentration of deoxyadenosine (A) nucleotides equals that of thymidine (T) nucleotides , while the concentration of deoxyguanosine (G) nucleotides equals that of deoxycytidine (C) nucleotides
, led Watson, Crick, and Wilkins to propose in the early 1950s a model of a double-stranded DNA molecule. The model they proposed is depicted in Figure 34–2. The two strands of this double-stranded helix are held in register by both hydrogen bonds between the purine and pyrimidine bases of the respective linear molecules and by van der Waals and hydrophobic interactions between the stacked adjacent base pairs. The pairings between the purine and pyrimidine nucleotides on the opposite strands are very specific and are dependent upon hydrogen bonding of A with T and G with C (Figure 34–2).
FIGURE 34–1 A segment of one strand of a DNA molecule in which the purine and pyrimidine bases guanine (G), cytosine (C), thymine (T), and adenine (A) are held together by a phosphodiester backbone between 2′-deoxyribosyl moieties attached to the nucleobases by an N-glycosidic bond. Note that the backbone has a polarity (ie, a direction). Convention dictates that a single-stranded DNA sequence is written in the 5′ to 3′ direction (ie, pGpCpTpA, where G, C, T, and A represent the four bases and p represents the interconnecting phosphates).
FIGURE 34–2 A diagrammatic representation of the Watson and Crick model of the double-helical structure of the B form of DNA. The horizontal arrow indicates the width of the double helix (20 Å), and the vertical arrow indicates the distance spanned by one complete turn of the double helix (34 Å). One turn of B-DNA includes 10 base pairs (bp), so the rise is 3.4 Å per bp. The central axis of the double helix is indicated by the vertical rod. The short arrows designate the polarity of the antiparallel strands. The major and minor grooves are depicted. (A, adenine; C, cytosine; G, guanine; P, phosphate; S, sugar [deoxyribose]; T, thymine.) Hydrogen bonds between A/T and G/C bases indicated by short, red, horizontal lines.
This common form of DNA is said to be right-handed because as one looks down the double helix, the base residues form a spiral in a clockwise direction. In the double-stranded molecule, restrictions imposed by the rotation about the phosphodiester bond, the favored anticonfiguration of the glycosidic bond (Figure 32–5), and the predominant tautomers (see Figure 32–2) of the four bases (A, G, T, and C) allow A to pair only with T and G only with C, as depicted in Figure 34–3. This base-pairing restriction explains the earlier observation that in a double-stranded DNA molecule the content of A equals that of T and the content of G equals that of C. The two strands of the double-helical molecule, each of which possesses a polarity, are antiparallel; that is, one strand runs in the 5′ to 3′ direction and the other in the 3′ to 5′ direction. In the double-stranded DNA molecules, the genetic information resides in the sequence of nucleotides on one strand, the template strand. This is the strand of DNA that is copied during ribonucleic acid (RNA) synthesis. It is sometimes referred to as the noncoding strand. The opposite strand is considered the coding strand because it matches the sequence of the RNA transcript (but containing uracil in place of thymine; see Figure 34–8) that encodes the protein.
FIGURE 34–3 DNA base pairing between adenine and thymine involves the formation of two hydrogen bonds. Three such bonds form between cytidine and guanine. The broken lines represent hydrogen bonds.
The two strands, in which opposing bases are held together by interstrand hydrogen bonds, wind around a central axis in the form of a double helix. In the test tube, double-stranded DNA can exist in at least six forms (A-E and Z). The B form is usually found under physiologic conditions (low salt, high degree of hydration). A single turn of B-DNA about the long axis of the molecule contains 10 bp. The distance spanned by one turn of B-DNA is 3.4 nm (34 Å). The width (helical diameter) of the double helix in B-DNA is 2 nm (20 Å).
As depicted in Figure 34–3, three hydrogen bonds, formed by hydrogen bonded to electronegative N or O atoms, hold the deoxyguanosine nucleotide to the deoxycytidine nucleotide, whereas the other pair, the A-T pair, is held together by two hydrogen bonds. Thus, the G-C bonds are more resistant to denaturation, or strand separation, termed “melting,” than A-T-rich regions of DNA.
The Denaturation of DNA Is Used to Analyze Its Structure
The double-stranded structure of DNA can be separated into two component strands in solution by increasing the temperature or decreasing the salt concentration. Not only do the two stacks of bases pull apart, but the bases themselves unstack while still connected in the polymer by the phosphodiester backbone. Concomitant with this denaturation of the DNA molecule is an increase in the optical absorbance of the purine and pyrimidine bases—a phenomenon referred to as hyperchromicity of denaturation. Because of the stacking of the bases and the hydrogen bonding between the stacks, the double-stranded DNA molecule exhibits properties of a rigid rod and in solution is a viscous material that loses its viscosity upon denaturation.
The strands of a given molecule of DNA separate over a temperature range. The midpoint is called the melting temperature, or Tm. The Tm is influenced by the base composition of the DNA and by the salt concentration of the solution. DNA rich in G-C pairs, which have three hydrogen bonds, melts at a higher temperature than that rich in A-T pairs, which have two hydrogen bonds. A 10-fold increase of monovalent cation concentration increases the Tm by 16.6°C. The organic solvent formamide, which is commonly used in recombinant DNA experiments, destabilizes hydrogen bonding between bases, thereby lowering the Tm. Formamide addition allows the strands of DNA or DNA-RNA hybrids to be separated at much lower temperatures and minimizes the phosphodiester bond breakage that can occur at higher temperatures.
Renaturation of DNA Requires Base Pair Matching
Importantly, separated strands of DNA will renature or reassociate when appropriate physiologic temperature and salt conditions are achieved; this reannealing process is often referred to as hybridization. The rate of reassociation depends upon the concentration of the complementary strands. Reassociation of the two complementary DNA strands of a chromosome after transcription is a physiologic example of renaturation (see below). At a given temperature and salt concentration, a particular nucleic acid strand will associate tightly only with a complementary strand. Hybrid molecules will also form under appropriate conditions. For example, DNA will form a hybrid with a complementary DNA (cDNA) or with a cognate messenger RNA (mRNA; see below). When hybridization is combined with gel electrophoresis techniques that separate nucleic acids by size coupled with radioactive or fluorescent probe labeling to provide a detectable signal, the resulting analytic techniques are called Southern (DNA/DNA) and Northern (RNA-DNA) blotting, respectively. These procedures allow for very distinct, high-sensitivity identification of specific nucleic acid species from complex mixtures of DNA or RNA (see Chapter 39).
There Are Grooves in the DNA Molecule
Examination of the model depicted in Figure 34–2 reveals a major groove and a minor groove winding along the molecule parallel to the phosphodiester backbones. In these grooves, proteins can interact specifically with exposed atoms of the nucleotides (via specific hydrophobic and ionic interactions) thereby recognizing and binding to specific nucleotide sequences as well as the unique shapes formed therefrom. Binding usually occurs without disrupting the base pairing of the double-helical DNA molecule. As discussed in Chapters 36 and 38. regulatory proteins control the expression of specific genes via such interactions.
DNA Exists in Relaxed & Supercoiled Forms
In some organisms such as bacteria, bacteriophages, many DNA-containing animal viruses, as well as organelles such as mitochondria (see Figure 35–8
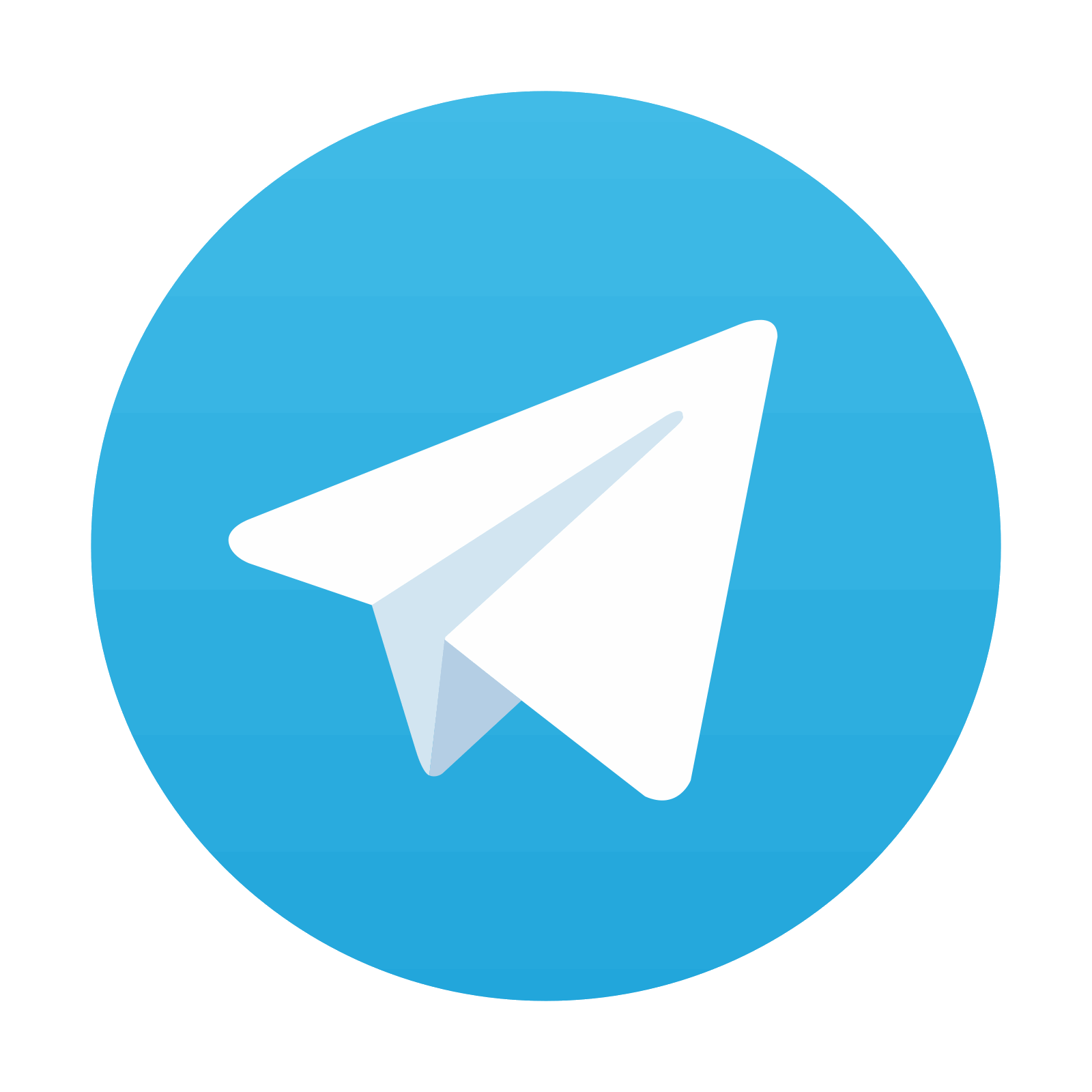
Stay updated, free articles. Join our Telegram channel
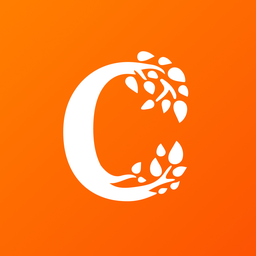
Full access? Get Clinical Tree
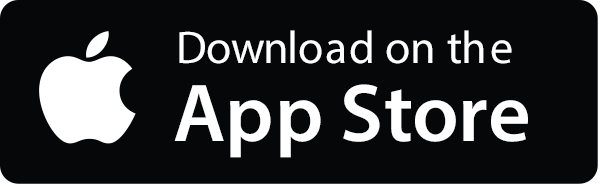
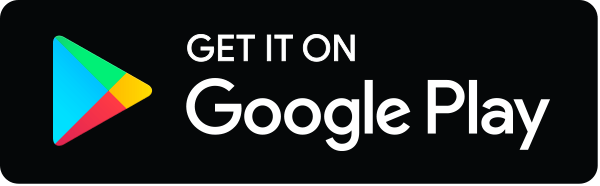