Noroviruses (NoVs) are a leading cause of sporadic and epidemic gastroenteritis across all age groups worldwide. The increasing recognition of the burden of disease caused by these viruses and their public health impact underscore the need for well tolerated and effective vaccines. In the absence of a completely permissive cell culture system, there is limited possibility of developing live-attenuated or killed NoV vaccines. Despite these challenges, many advances have been made in vaccine development in recent years. A number of vaccine candidates have been evaluated including the production of virus-like particles (VLPs) that are morphologically and antigenically identical to particles of the infectious virus. NoV VLPs appear to be attractive vaccine candidates, and the advances in the area of these vaccines are highly promising. This chapter summarizes data on immune correlates of protection against NoVs, preclinical and clinical studies with NoV candidate vaccines, and discusses the challenges remaining in the field of NoV vaccine development.
Norovirus Vaccine Development
Abstract
Keywords
1. Background
2. Immune correlates

Panels A–C show blocking antibody responses following infection with GI.1 virus while panels D–F show blocking antibody responses following vaccination with GI.1.and GII.4c VLPs. Y-axis in panels A, B, D, E represents 50% percent blocking titers (BT50 for infection, EC50 for vaccination as defined in the original studies describing these results) and is defined as the serum titer at which 50% of the VLP-carbohydrate interaction is blocked when compared to the positive control. The geometric mean titers (GMT) of blocking antibodies to GI VLPs following infection and vaccination are seen in Panels A, D respectively. Panels B, E show geometric mean titers (GMT) of blocking antibodies to GII VLPs following infection and vaccination. Shaded cells in panels C and F represent individuals who showed a >fourfold change in blocking antibody response post infection and vaccination, respectively. The response to each NoV genotype or variant is represented by a different color.
Table 3.6.1
Immune Correlates of Protection
Correlate | Outcome | References |
Preexposure | ||
Serum histo-blood group antigen (HBGA)-blocking antibody | Illness | Reeck et al. (2010), Atmar et al. (2011), Atmar et al. (2015) |
Infection | Atmar et al. (2011), Atmar et al. (2015) | |
Serum hemagglutination inhibition (HAI) antibody | Illness | Czako et al. (2012) |
Salivary IgA antibody | Illness | Ramani et al. (2015) |
Memory B cells | Illness | Ramani et al. (2015) |
Fecal IgA antibody | Peak virus shedding | Ramani et al. (2015) |
Postexposure | ||
Rapid salivary IgA response | Infection | Lindesmith et al. (2003) |
Day 7 fecal IgA response | Duration of virus shedding | Ramani et al. (2015) |
3. Preclinical studies with norovirus vaccine candidates
Table 3.6.2
Norovirus Vaccine Candidates
Antigen | Expression system | References |
Virus-like particles | Baculovirus | Jiang et al. (1992) |
Pichia (yeast) | Xia et al. (2007) | |
Venezuelan equine encephalitis virus replicon | Harrington et al. (2002) | |
Plants (tobacco, potato, tomato) | Mason et al. (1996), Zhang et al. (2006) | |
Recombinant adenovirus | Guo et al. (2008) | |
P particles | E. coli | Tan and Jiang (2005) |
Table 3.6.3
Select Preclinical Studies of NoV Vaccine Candidates
Immunogen | Animal | Route | Adjuvant | Findings | References |
Baculovirus-expressed GI.1/Norwalk VLPs | Mice | Oral gavage | +/– cholera toxin | Oral administration of unadjuvanted vaccine induced systemic and mucosal immune responses, and use of adjuvant augmented responses | Ball et al. (1998) |
Baculovirus-expressed GI.1/Norwalk VLPs | Mice | Intranasal (IN) versus oral gavage | +/− E. coli mutant (R192G) labile toxin (LT) | Intranasal administration of unadjuvanted vaccine induced systemic and mucosal immune responses, and use of adjuvant augmented responses at lower dosages than following oral gavage | Guerrero et al. (2001) |
Baculovirus-expressed GI.1/Norwalk VLPs | Mice | Intranasal versus oral gavage | +/− mutant (E29H) cholera toxin | Adjuvant enhanced cellular and serological immune responses, and responses were higher following IN administration compared to the oral route | Periwal et al. (2003) |
Baculovirus-expressed GII.4/Dijon VLPs | Mice | Intranasal versus oral gavage | +/– E. coli wild type (wt) or mutant (R192G) labile toxin | Adjuvant enhanced cellular and serological immune responses, and responses were higher following IN administration compared to the oral route. Responses were similar when wt LT or mutant LT were used as adjuvant | Nicollier-Jamot et al. (2004) |
Baculovirus-expressed GII.4/HS66 VLPs | Gnotobiotic pigs | Oral and intranasal | E. coli mutant (R192G) labile toxin or ISCOM matrix | Both adjuvanted vaccines induced serological and cellular immune responses and were associated with decreased viral shedding and diarrhea after virus challenge compared to control animals | Souza et al. (2007) |
Baculovirus-expressed GI.1/Norwalk versus GII.4/MD145 VLPs | Chimpanzees | Intramuscular (IM) | Aluminum hydroxide | Seroresponses occurred after vaccination; only immunization with the homologous antigen protected against infection following intravenous challenge with Norwalk virus | Bok et al. (2011) |
GI.1/Norwalk VLPs—tobacco plant extracts and potato expressing VLPs | Mice | Oral gavage | +/– cholera toxin | Plant-expressed VLPs were immunogenic | Mason et al. (1996) |
GII.4/VA387 VLPs—Pichia pastoris-expressed raw extract | Mice | Oral gavage or intramuscular | Ribi adjuvant with IM injection | Yeast-expressed VLPs immunogenic after IM delivery and when orally-delivered, induced serum and fecal antibody responses, including production of serum HBGA-blocking antibody | Xia et al. (2007) |
Venezuelan equine encephalitis (VEE) replicons and VEE replicon-expressed GI.1/Norwalk VLPs | Mice | Footpad inoculation or oral gavage | None | Serum antibody responses were induced by both routes of immunization, but the replicon induced higher antibody levels than obtained following oral VLP administration | Harrington et al. (2002) |
VEE replicons expressing GI.1/Norwalk, GII.1/Hawaii, GII.2/Snow Mountain, GII.4/Lordsdale VLPs | Mice | Footpad inoculation | None | Monovalent preparations induced only homotypic HBGA-blocking antibody responses, while a trivalent (GI.1, GII.1, GII.2) preparation induced a heterotypic response to a fourth genotype (GII.4), although to a lesser degree than when the GII.4 strain was included in a vaccination regimen (as monovalent or quadrivalent) | LoBue et al. (2006) |
GII.4/VA387 P particles expressed in E. coli and yeast | Mice | Intranasal | None | P particle vaccine had immunogenicity similar to that achieved using VLPs as the immunogen, including induction of strain-specific HBGA-blocking antibody | Tan et al. (2008) |
E. coli-expressed GII.4/VA387 P particles and chimeric P particles with rotavirus VP8 | Mice | Intranasal, subcutaneous | Freund’s adjuvant (subcutaneous only) | The chimera enhanced responses to VP8 compared to free VP8 delivered IN; it induced immune responses that provided protection against rotavirus challenge in a mouse model; and it induced homotypic norovirus HBGA-blocking antibodies | Tan et al. (2011) |
GII.4/VA387 baculovirus-expressed VLPs and E. coli-expressed P particles and P dimers | Mice | Intranasal | None | VLPs and P particles induced higher levels of antibody and CD4 T cellular immune responses compared to the P dimers | Fang et al. (2013) |
Baculovirus-expressed GII.4/1999 VLPs; E. coli-expressed GII.4/1999 P particles | Mice | Intramuscular, intradermal | None | VLPs induced higher serum antibody levels, heterotypic ELISA antibody responses, more balanced Th1/Th2 antibody response, and IFN-gamma-expressing cellular responses compared to P particles | Tamminen et al. (2012) |
Baculovirus-expressed GI.3, GII.4/1999, rotavirus rVP6 | Mice | Intramuscular | None | Trivalent vaccine induced homotypic and heterotypic serum antibody responses, mucosal antibody responses and T cell responses | Tamminen et al. (2013) |
E. coli-expressed GII.4/VA387 P dimers or fusion proteins with hepatitis E virus P domain dimers | Mice | Intranasal | None | Immunization with the fusion protein induced hepatitis E virus neutralizing antibodies and homotypic norovirus HBGA-blocking antibodies | Wang et al. (2014) |
4. Clinical studies
Table 3.6.4
Summary of Immunogenicity From Clinical Studies of Norovirus Vaccine Candidates
Vaccine (Manufacturer) | Route | Dosage (Adjuvant) | Schedule | Number of Subjects (Age range) | Peak Serum IgG GMFR Reported (95% CI) | Peak Serum IgA GMFR Reported (95% CI) | Serum HAI or HBGA-blocking antibody GMFR Reported (95% CI) | References |
GI.1/Norwalk (Baylor College of Medicine) | Oral | 100 mcg (none) | 2 doses 3 wks apart | 5 (18–46 yrs) | 5.3 (1.3–22.3) | 3.0 (1.4–6.5) | ND | Ball et al. (1999) |
250 mcg (none) | 2 doses 3 wks apart | 15 (18–46 yrs) | 8.8 (5.4–14.2) | 4.4 (2.1–9.2) | ND | |||
GI.1/Norwalk (Baylor College of Medicine) | Oral | 250 mcg (none) | 2 doses 3 wks apart | 10 (18–40 yrs) | ∼13a (NR) | NR | ND | Tacket et al. (2003) |
500 mcg (none) | 2 doses 3 wks apart | 10 (18–40 yrs) | ∼13a (NR) | NR | ND | |||
2000 mcg (none) | 2 doses 3 wks apart | 10 (18–40 yrs) | ∼18a (NR) | NR | ND | |||
GI.1/Norwalk in potato (Boyce Thompson Institute for Plant Research) | Oral | 150 g potato containing 215–751 mcg VLP | 2 doses 3 wks apart | 10 (adult) | 8a (not calculable) | ND | ND | Tacket et al. (2000) |
150 g potato containing 215–751 mcg VLP | 3 doses on days 0, 7, and 21 | 10 (adult) | 13.3a (NR) | ND | ND | |||
GI.1/Norwalk (Ligocyte/Takeda) | Intranasal | 5 mcg (chitosan, MPL) | 2 doses 3 wks apart | 5 (18–49 yrs) | 0.9 (NR) | 1.2 (NR) | ND | El-Kamary et al. (2010) |
15 mcg (chitosan, MPL) | 2 doses 3 wks apart | 5 (18–49 yrs) | 1.9 (NR) | 2.5 (NR) | ND | |||
50 mcg (chitosan, MPL) | 2 doses 3 wks apart | 9 (18–49 yrs) | 4.7 (NR) | 4.5 (NR) | ND | |||
50 mcg (chitosan, MPL) | 2 doses 3 wks apart | 18 (18–49 yrs) | 4.6 (2.5–8.6) | 7.6 (4.2–13.8) | 4.0 (2.0–7.9)—HAI | |||
100 mcg (chitosan, MPL) | 2 doses 3 wks apart | 19 (18–49 yrs) | 4.8 (3.2–7.1) | 9.1 (4.7–17.6) | 9.1 (4.0–20.7)—HAI | |||
GI.1/Norwalk (Ligocyte/Takeda) | Intranasal | 100 mcg (chitosan, MPL) | 2 doses 3 wks apart | 37 (18–50 yrs) | 4.5 (3.1–6.5) | 7.5 (4.6–12.2) | 3.6 (2.8–4.7) HBGA-blocking | Atmar et al. (2011) |
GI.1/Norwalk and GII.4/consensus (Ligocyte/Takeda) | Intramuscular | 5 mcg each (MPL, aluminum hydroxide) | 2 doses 4 wks apart | 10 (18–49 yrs) | NR | NR | NR | Treanor et al. (2014) |
15 mcg each (MPL, aluminum hydroxide) | 2 doses 4 wks apart | 10 (18–49 yrs) | NR | NR | NR | |||
150 mcg each (MPL, aluminum hydroxide) | 2 doses 4 wks apart | 9 (18–49 yrs) | NR | NR | NR | |||
50 mcg each (MPL, aluminum hydroxide) | 2 doses 4 wks apart | 18 (18–49 yrs) | GI.1: ∼29 (NR) GII.4: ∼11 (NR) | GI.1: ∼39 (NR) GII.4: ∼14 (NR) | GI.1: 16 (NR) GII.4: 10 (NR) HBGA-blocking | |||
50 mcg each (MPL, aluminum hydroxide) | 2 doses 4 wks apart | 9 (50–64 yrs) | GI.1: ∼57 (NR) GII.4: ∼13 (NR) | GI.1: ∼45 (NR) GII.4: ∼10 (NR) | GI.1: 16 (NR) GII.4: 11 (NR) HBGA-blocking | |||
50 mcg each (MPL, aluminum hydroxide) | 2 doses 4 wks apart | 10 (65–85 yrs) | GI.1: (∼13 (NR) GII.4: ∼6 (NR) | GI.1: ∼42 (NR) GII.4: ∼5 (NR) | GI.1: 16 (NR) GII.4: 6 (NR) HBGA-blocking | |||
GI.1/Norwalk and GII.4/consensus (Ligocyte/Takeda) | Intramuscular | 50 mcg each (MPL, aluminum hydroxide) | 2 doses 4 wks apart | 49 (18–50 yrs) | GI.1: 27.8 (19.2, 40.2) GII.4: 8.8 (6.5, 11.9) | GI.1: 17.5 (11.9, 25.8) GII.4: 9.3 (6.7, 12.7) | GI.1: 31.6 (24.6, 40.5) GII.4: 7.8 (5.9, 10.2) HBGA-blocking | Bernstein et al. (2015), Atmar et al. (2015) |
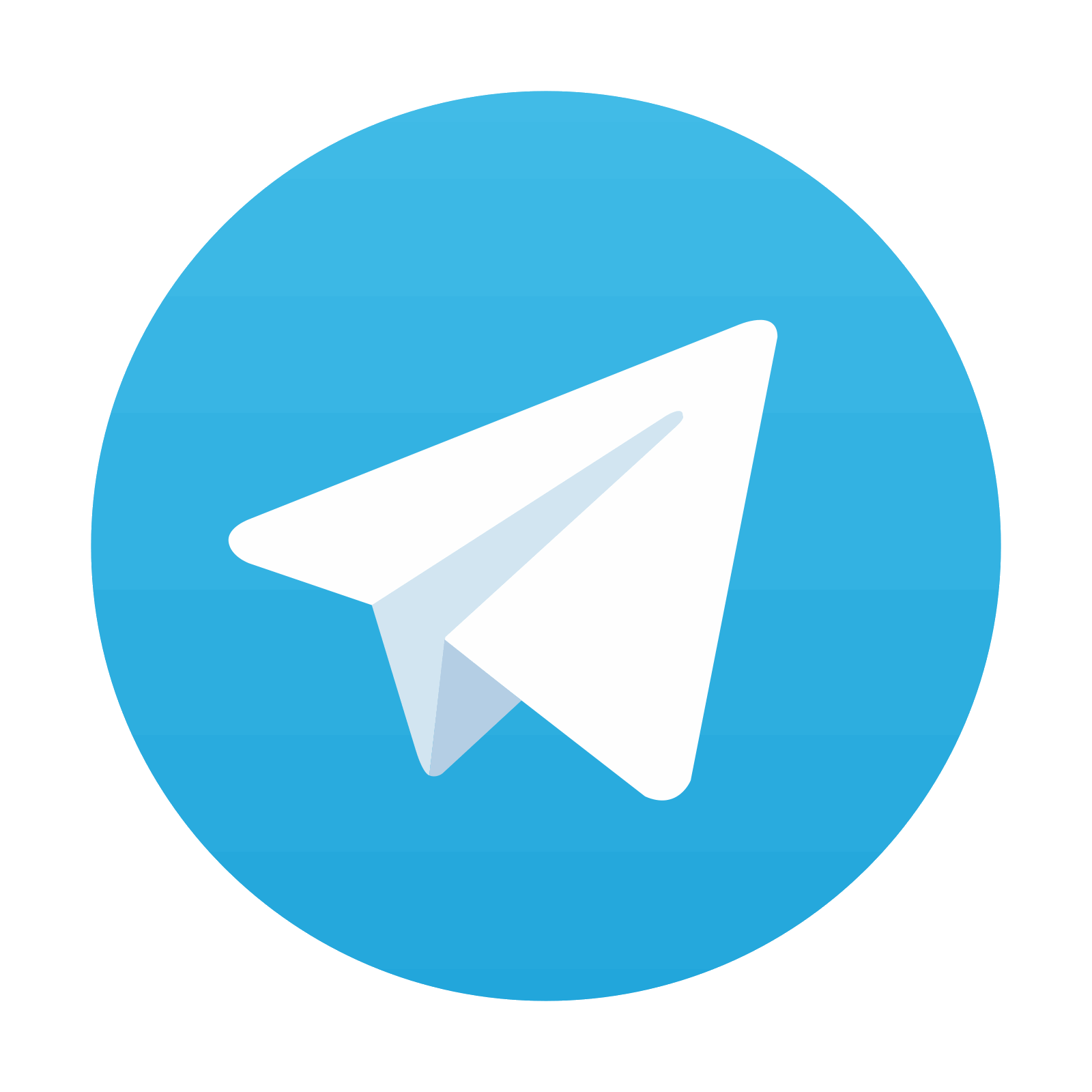
Stay updated, free articles. Join our Telegram channel
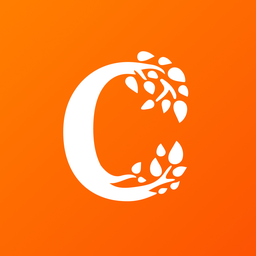
Full access? Get Clinical Tree
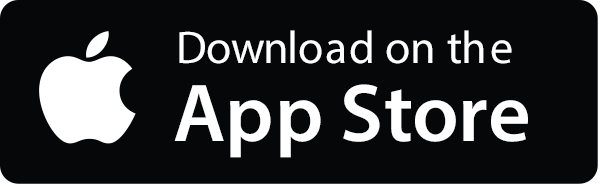
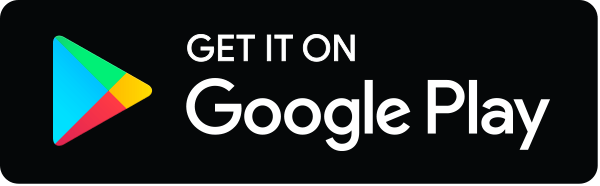