5
Neurotransmission
Chapter 5, Neurotransmission is a compilation of Chapter 8 Neurotransmission: The Autonomic and Somatic Motor Nervous Systems, and Chapter 14 Neurotransmission and the Central Nervous System in Goodman & Gilman’s The Pharmacological Basis of Therapeutics, 12th Edition. An understanding of the material in these chapters will be helpful in following the material presented in this chapter. In addition to the material presented here, the 12th Edition includes:
• A detailed discussion of the peripheral and central nervous systems (CNS)
• An intricate discussion of the neurotransmitters in the peripheral and CNS, their synthesis, storage, mechanisms of release and termination of effect
• Details of the cholinergic, adrenergic, and somatic nervous systems that are also addressed in Chapters 6 and 7 of this book
• A detailed discussion of chemical transmission of impulses in the CNS
LEARNING OBJECTIVES
Understand the characteristics of the parasympathetic, sympathetic, and enteric nervous systems.
Understand the difference between the autonomic and somatic nervous systems.
Know the predominant transmitters at ganglionic sites in the parasympathetic and sympathetic nerves.
Know the transmitters and their target receptors in parasympathetic and sympathetic nervous system.
Know the effect that agonist and antagonists have at each target receptor.
Understand the blood-brain barrier (BBB).
Understand the intricacies of chemical transmission within the CNS and how the interaction of central neurons control the pharmacological effects of drugs acting in the brain.
DRUGS INCLUDED IN THIS CHAPTER
The pharmacology of specific drugs is not included in this chapter. The drugs presented in the cases are used as examples to illustrate various aspects of neurotransmission; the specific pharmacology of these drugs is presented in the subsequent chapters of this section.
A 47-year-old woman is given a drug to treat her overactive bladder. She is told that the drug is similar to atropine and that it will decrease her frequency of urination. She is cautioned to be aware of the possibility of dry eyes, dry mouth, blurred vision, constipation, drowsiness, dizziness, and confusion.
a. What are the divisions of the peripheral autonomic nervous system that describe the diverse actions of this drug?
The efferent nerves of the involuntary (autonomic) nervous system supply all innervated structures of the body except skeletal muscle, which is served by the somatic nerves (see Figures 5-1 and 5-2). The peripheral autonomic nervous system is composed of the sympathetic, parasympathetic, and enteric divisions (see Side Bar DIVISIONS OF THE PERIPHERAL AUTONOMIC NERVOUS SYSTEM).
FIGURE 5-1 The autonomic nervous system. Schematic representation of the autonomic nerves and effector organs based on chemical mediation of nerve impulses. Blue, cholinergic; grey, adrenergic; dotted blue, visceral afferent; solid lines, preganglionic; broken lines, postganglionic. In the rectangle at the right are shown the finer details of the ramifications of adrenergic fibers at any 1 segment of the spinal cord, the path of the visceral afferent nerves, the cholinergic nature of somatic motor nerves to skeletal muscle, and the presumed cholinergic nature of the vasodilator fibers in the dorsal roots of the spinal nerves. The asterisk (*) indicates that it is not known whether these vasodilator fibers are motor or sensory or where their cell bodies are situated.
FIGURE 5-2 Schematic representation of the somatic motor nerves and the efferent nerves of the autonomic nervous system. The principal neurotransmitters, acetylcholine(ACh) and norepinephrine (NE), are shown in grey. The receptors for these transmitters, nicotinic (N) and muscarinic (M) cholinergic receptors, α and β adrenergic receptors are shown in grey rectangles to the right. The somatic nerves innervate skeletal muscle directly without a ganglionic relay. The autonomic nerves innervate smooth muscles, cardiac tissue, and glands. Both parasympathetic and sympathetic systems have ganglia where ACh is the transmitter of the preganglionic fibers; ACh acts on nicotinic receptors on the postganglionic nerves. ACh is also the neurotransmitter at cells of the adrenal medulla, where it acts on nicotinic ACh receptors to cause release of the catecholamines epinephrine (Epi) and NE into the circulation. Epi represents ~80% of the released catecholamines. ACh is the predominant neurotransmitter of postganglionic parasympathetic nerves and acts on muscarinic receptors. NE is the principal neurotransmitter of postganglionic sympathetic nerves, acting on α or β adrenergic receptors. Note that somatic nerves form a specialized synaptic junction, termed the motor end plate. Autonomic nerves form a more diffuse pattern with multiple synaptic sites. The ganglia in the parasympathetic system are near or within the organ being innervated with generally a one-to-one relationship between pre- and postganglionic fibers. In the sympathetic system, the ganglia are generally far from the effector cells (eg, within the sympathetic chain ganglia). Preganglionic sympathetic fibers may make contact with a large number of postganglionic fibers.
b. Why does this drug have such a broad constellation of side effects involving so many different organs?
Atropine is a drug that blocks cholinergic muscarinic receptors located on the membranes of many effector organs (see Figure 5-1 and Table 5-1), and it blocks all the peripheral actions of acetylcholine, the parasympathetic neurotransmitter (see Chapter 6). Thus, it is easy to see why its side effects involve so many organs. Although atropine has minimal effects on the CNS, there are definitely muscarinic receptors in the brain and their antagonism may lead to drowsiness and confusion.
TABLE 5-1 Responses of Effector Organs to Autonomic Nerve Impulses
c. What is the enteric nervous system?
The processes of mixing, propulsion, and absorption of nutrients in the GI tract are controlled locally through a restricted part of the peripheral nervous system called the enteric nervous system (ENS; see Chapter 33). The ENS actually comprises components of the sympathetic and parasympathetic nervous systems and has sensory nerve connections through the spinal and nodose ganglia.
d. What are the differences between the parasympathetic and sympathetic nervous systems?
The sympathetic system is distributed to effectors throughout the body, whereas parasympathetic distribution is much more limited (see Figure 5-1). The ganglia in the parasympathetic system are near or within the organ being innervated with generally a one-to-one relationship between pre- and postganglionic fibers (see Figure 5-2). In the sympathetic system the ganglia are generally far from the effector cells. Preganglionic sympathetic fibers may make contact with a large number of postganglionic fibers.
In contrast to the parasympathetic and sympathetic nervous systems, somatic nerves innervate skeletal muscle directly without a ganglionic relay (see Case 5-2).
Acetylcholine (ACh) is the predominant neurotransmitter of postganglionic parasympathetic nerves and acts on muscarinic receptors. Norepinephrine (NE) is the principal neurotransmitter of postganglionic sympathetic nerves acting on α or β adrenergic receptors.
Both parasympathetic and sympathetic systems have ganglia where ACh is the transmitter of the preganglionic fibers; ACh acts on nicotinic receptors on the postganglionic nerves. ACh is also the transmitter at cells of the adrenal medulla, where it acts on nicotinic receptors to cause release of epinephrine and norepinephrine into the circulation.
DIVISIONS OF THE PERIPHERAL AUTONOMIC NERVOUS SYSTEM
• Sympathetic nervous system
• Parasympathetic nervous system
• Enteric nervous system
STEPS INVOLVED IN JUNCTIONAL TRANSMISSION
• Transmitter synthesis
• Transmitter storage
• Transmitter release
• Transmitter recognition and binding by target receptor
• Termination of action
A 35-year-old man is undergoing abdominal surgery. The anesthesiologist explains in a presurgery meeting with the patient that a neuromuscular blocking drug will be administered to cause complete muscle relaxation prior to the initiation of surgery.
a. What is the difference between the autonomic nervous system that innervates many visceral organs and the somatic nervous system that innervates skeletal muscle?
The somatic nerves innervate skeletal muscle directly without a ganglion relay (see Figure 5-2). At each neuromuscular junction, the axon terminal loses its myelin sheath and forms a terminal aborization that lies in apposition to a specialized surface of the muscle membrane termed the motor end plate (see Chapter 6).
b. What are the steps involved in the transmission of a nerve impulse?
The steps involved in excitatory and inhibitory neurotransmission are depicted schematically in Figure 5-3. The steps involved in the junctional transmission of the nerve impulse are outlined in the Side Bar STEPS INVOLVED IN JUNCTIONAL TRANSMISSION, the details of which are characteristic of a specific nerve transmission system, that is, parasympathetic, sympathetic, somatic, and in the CNS.
FIGURE 5-3 Steps involved in excitatory and inhibitory neurotransmission. 1. The nerve action potential (AP) consists of a transient self-propagated reversal of charge on the axonal membrane. (The internal potential Ei goes from a negative value, through zero potential, to a slightly positive value primarily through increases in Na+ permeability and then returns to resting values by an increase in K+ permeability.) When the AP arrives at the presynaptic terminal, it initiates release of the excitatory or inhibitory transmitter. Depolarization at the nerve ending and entry of Ca2+ initiate docking and then fusion of the synaptic vesicle with the membrane of the nerve ending. Docked and fused vesicles are shown. 2. Combination of the excitatory transmitter with postsynaptic receptors produces a localized depolarization, the excitatory postsynaptic potential (EPSP), through an increase in permeability to cations, most notably Na+. The inhibitory transmitter causes a selective increase in permeability to K+ or Cl–, resulting in a localized hyperpolarization, the inhibitory postsynaptic potential (IPSP). 3. The EPSP initiates a conducted AP in the postsynaptic neuron; this can be prevented, however, by the hyperpolarization induced by a concurrent IPSP. The transmitter is dissipated by enzymatic destruction, by reuptake into the presynaptic terminal or adjacent glial cells, or by diffusion. Depolarization of the postsynaptic membrane can permit Ca2+ entry if voltage-gated Ca2+ channels are present. (Reproduced with permission from Brunton L, Parker K, Blumenthal D, Buxton I (eds). Goodman & Gilman’s Manual of Pharmacology and Therapeutics. New York: McGraw-Hill, 2008, p 94. Copyright © 2008 by The McGraw-Hill Companies, Inc. All rights reserved.)
Transmitter synthesis: Small molecules like ACh and NE (see Side Bar NEUROTRANSMITTERS for a list of neurotransmitters) are synthesized in nerve terminals; peptides are synthesized in cell bodies and transported to nerve terminals.
Transmitter storage: Synaptic vesicles store transmitters, often in association with various proteins and frequently with ATP.
Transmitter release: Release of transmitter occurs by exocytosis. Depolarization results in an influx of Ca++, which in turn appears to bind proteins called synaptotagmins. The storage vesicles dock and then fuse with scaffolding proteins on the presynaptic membrane, which is followed by exocytotic release of their contents into the synaptic cleft (see Figure 5-3).
Transmitter recognition: Receptors exist on postsynaptic cells, which recognize the transmitter (see Side Bar NEUROTRANSMITTERS). Binding of a neurotransmitter to its receptor initiates a signal transduction event (see Figures 5-4 and 5-6).
FIGURE 5-4 Structural similarities of voltage-dependent Na+, Ca2+, and K+ channels. A. The α subunit in both Ca2+ and Na+ channels contains 4 subunits, each with 6 transmembrane hydrophobic domains. The hydrophobic regions that connect segments 5 and 6 in each domain form the pore of the channel. Segment 4 in each domain includes the voltage-sensor. (Adapted with permission from Catterall W. From ionic currents to molecular mechanisms: The structure and function of voltage-gated sodium channels. Neuron, 2000, 26:13–25. Copyright © Elsevier.) B. The Ca2+ channel also requires several auxiliary small proteins (α2, β, γ, and δ). The α2 and δ subunits are linked by a disulfide bond. Regulatory subunits also exist for Na+ channels. C. Voltage-sensitive K+ channels (Kv) and the rapidly activating K+ channel (KA) share a similar putative hexaspanning structure similar in overall configuration to 1 repeat unit within the Na+ and Ca2+ channel structure, while the inwardly rectifying K+ channel protein (Kir) retains the general configuration of just loops 5 and 6. Regulatory β subunits (cystosolic) can alter Kv channel functions. Channels of these 2 overall motifs can form heteromultimers.
FIGURE 5-5 Predicted 3-D structure of a ligand-gated ion channel receptor in a postsynaptic membrane. A. These channels consist of a cylindrical membrane-embedded structure with a central pore. The second transmembrane domain (TM2) of each subunit lines the pore and bends inward to block ion flow through the channel. B. A highly conserved leucine residue (L) in the TM2 bend of each subunit is believed to protrude into the pore to form a tight hydrophobic ring, which may act as a barrier to the flow of hydrated ions across the channel. (Redrawn with permission from Nestler EJ, Hyman SE, Malenka RC (eds). Molecular Neuropharmacology, 2nd ed. New York: McGraw-Hill, 2009, p 174. Copyright © 2009 by The McGraw-Hill Companies, Inc. All rights reserved.)
FIGURE 5-6 General patterns of signal transduction in the brain. A. Neurotransmitter activation of a receptor that contains an integral ion channel. B. Neurotransmitter activation of G protein–coupled receptor. After activation, the βγ subunits of the G protein can directly regulate an ion channel (left), and the α subunit can activate second messenger-dependent signaling involving protein kinases and protein phosphatases, which can, in turn, affect ion channels and other neuronal processes (right). C. Neurotrophic factors promote receptor dimerization, which leads to activation of receptor protein tyrosine kinase activity and its sequelae. D. Steroid hormone activation of a cytoplasmic receptor. After the receptor-hormone complex forms, it enters the nucleus and regulates gene expression. (Redrawn with permission from Nestler EJ, Hyman SE, Malenka RC (eds). Molecular Neuropharmacology, 2nd ed. New York: McGraw-Hill, 2009, p 76. Copyright © 2009 by The McGraw-Hill Companies, Inc. All rights reserved.)
Termination of action: A variety of mechanisms terminate the action of synaptically released transmitter, including hydrolysis and reuptake into neurons by specific transporters (see Chapters 6 and 7 for specific examples).
NEUROTRANSMITTERS
c. What is the role of ion channels in the promulgation of the nerve impulse and controlling neuronal excitability?
Voltage-dependent ion channels provide for rapid changes in ion permeability along axons and within dendrites and for excitation-secretion coupling that releases neurotransmitters from presynaptic sites.
The electrical excitability of neurons is achieved through modification of ion channels in neuronal plasma membranes (see Figures 5-3 through 5-6). The relatively high extracellular concentration of Na+ (≈140 mM) compared to its concentration intracellularly (≈14 mM) means that increases in permeability to Na+ causes depolarization, ultimately leading to the generation of action potentials.
Increases in the concentration of intracellular Ca++ affects multiple processes in the cell and are critical to the release of neurotransmitters. Table 5-2 lists the various subtypes of Ca++ channels.
TABLE 5-2 Subtypes of Ca2+ Channela
The Cl– gradient across the plasma membrane explains the fact that activation of Cl– channels causes an inhibitory postsynaptic potential (IPSP) that dampens neuronal excitability and inactivation of these channels can lead to hyperexcitability. Figure 14-3 in Goodman and Gilman’s The Pharmacological Basis of Therapeutics, 12th Edition shows the structures of 3 families of Cl– channels.
A 6-year-old boy is accidentally exposed to a large oral dose of the pesticide malathione, a cholinesterase inhibitor.
a. Why are the symptoms that this boy might experience predictable?
Acetylcholinesterase catalyzes the hydrolysis of the neurotransmitter acetylcholine (ACh) (see Chapter 6). When the enzyme is inhibited, there is an abundance of ACh at the ganglion in parasympathetic and sympathetic fibers, at the parasympathetic effector organs, and at the somatic neuromuscular junctions (see Figure 5-2). Although poisoning by anticholinesterase agents is complex (see Chapter 6), knowing the organs innervated by parasympathetic, sympathetic, and somatic fibers can allow for the prediction of symptoms to be experienced by this boy (see Figure 5-1 and Table 5-1).
b. How are the actions of neurotransmitters terminated?
Figure 5-7 shows the various mechanisms by which neurotransmitters are terminated. Released neurotransmitter is inactivated by reuptake into the nerve terminal (dopamine, NE, GABA), by degradation (ACh, peptides), or by uptake and metabolism by glial cells in the CNS (glutamate).
FIGURE 5-7 Transmitter release, action, and inactivation. Depolarization opens voltage-dependent Ca2+ channels in the presynaptic nerve terminal. (1) The influx of Ca2+ during an action potential (AP) triggers (2) the exocytosis of small synaptic vesicles that store neurotransmitter (NT) involved in fast neurotransmission. Released neurotransmitter interacts with receptors in the postsynaptic membranes that either couple directly with ion channels (3) or act through second messengers, such as (4) GPCRs. Neurotransmitter receptors in the presynaptic nerve terminal membrane (5) can inhibit or enhance subsequent exocytosis. Released neurotransmitter is inactivated by reuptake into the nerve terminal by (6) a transport protein coupled to the Na+ gradient, for example, DA, NE, and GABA; by (7) degradation (ACh, peptides); or by (8) uptake and metabolism by glial cells (Glu). The synaptic vesicle membrane is recycled by (9) clathrin-mediated endocytosis. Neuropeptides and proteins are stored in (10) larger, dense core granules within the nerve terminal. These dense core granules are released from (11) sites distinct from active zones after repetitive stimulation.
RECEPTORS IN THE PERIPHERAL AND CENTRAL NERVOUS SYSTEMS
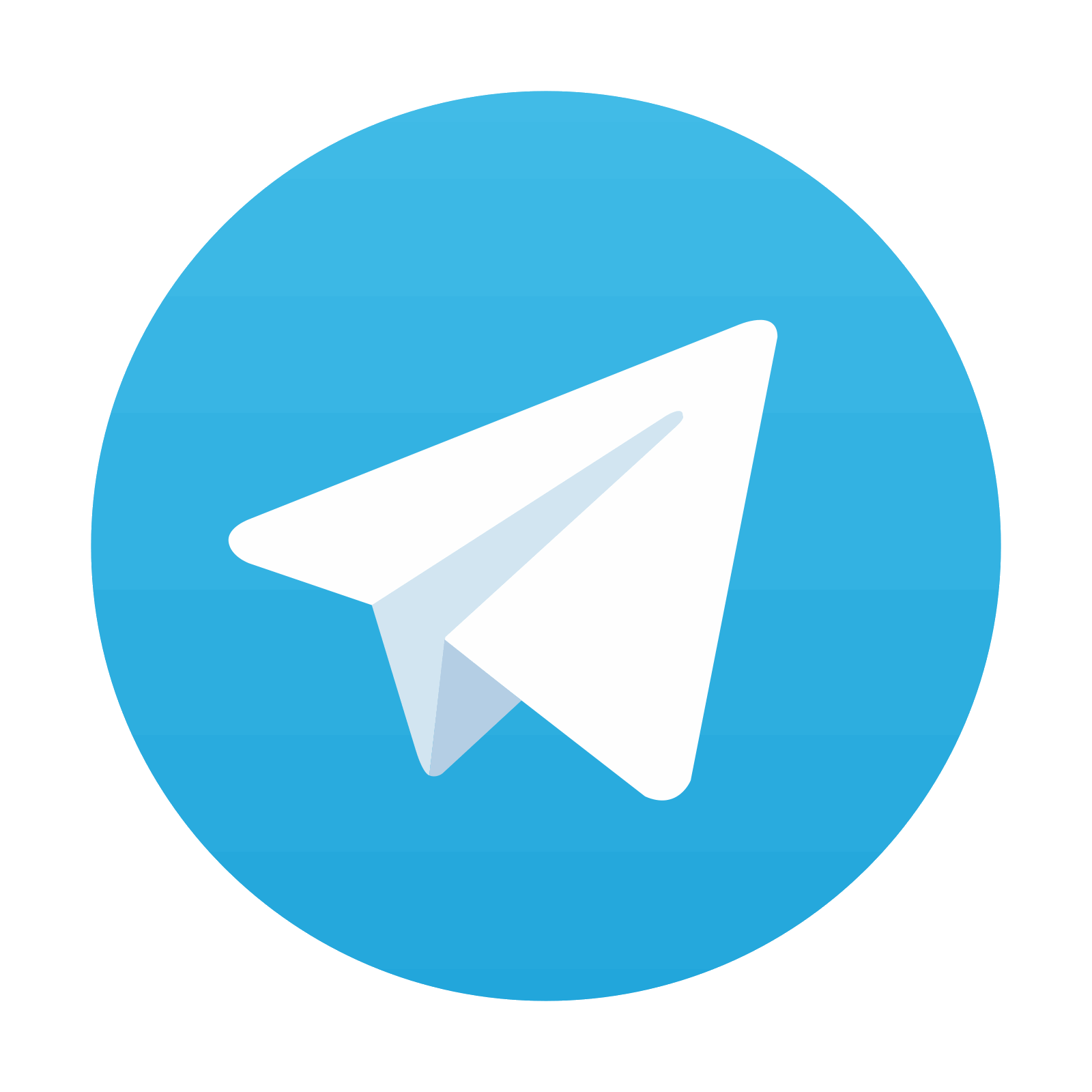
Stay updated, free articles. Join our Telegram channel
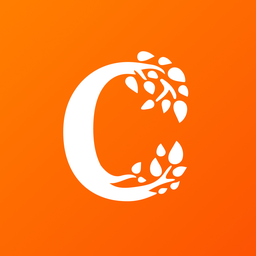
Full access? Get Clinical Tree
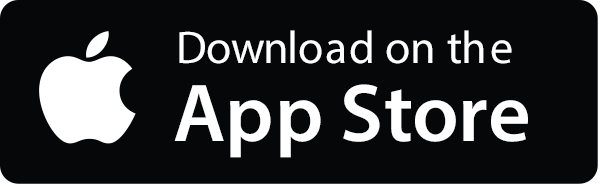
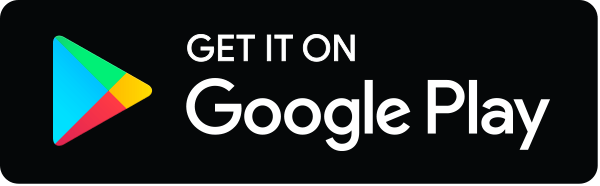